Proceedings of a Workshop
IN BRIEF | |
May 2018 |
|
![]() |
|
Direct Air Capture and Mineral Carbonation Approaches for Carbon Dioxide Removal and Reliable Sequestration Proceedings of a Workshop—in Brief |
Direct air capture (DAC) refers to a range of technologies that capture and concentrate carbon dioxide (CO2) from ambient air. These technologies can include chemical scrubbing processes that capture CO2 through absorption or adsorption separation processes. DAC can also refer to the process that involves rapid mineralization of CO2 at the Earth’s surface, termed mineral carbonation. When combined with reliable sequestration of the captured CO2, direct air capture and sequestration (DACS) has the potential to become a negative emission technology. Conventional carbon capture and sequestration (CCS), though comparable to DAC in the use of similar separation technologies, captures CO2 from a concentrated gas stream (such as flue gas from coal-fired power plants) and sequesters it.1
On October 5, 2017, the committee conducted a webinar-based panel discussion that explored the limitations, appropriate scale, and future costs (both capital and energy) of DAC technologies. Panelists described technological readiness, current research needs, and potential environmental impacts of DAC. The committee then held a workshop on October 24, 2017, in Irvine, CA, to examine the scientific questions relevant to developing a research and development plan for DAC moving forward, and to assess co-benefits, costs, and barriers to implementation of this technology at significant scales. This information-gathering workshop did not consider carbon utilization pathways in detail or other cross-cutting issues such as specific policy incentives or management.
___________________
1 National Research Council (NRC). 2015. Climate Intervention: Carbon Dioxide Removal and Reliable Sequestration. Washington, DC: The National Academies Press.
TABLE 1: Approaches to Direct Air Capture
Approach | Description | Current Capacity (tCO2 /year) |
---|---|---|
CCS | Chemical scrubbing processes that capture CO2 from a concentrated gas stream and sequestration | 35–40,000,000 |
DAC | Chemical scrubbing processes that capture CO2 through absorption or adsorption separation processes | |
Carbon capture using amine sorbents with a temperature swing for regeneration | 50,000 | |
|
Solid sorbent system coupled with a temperature swing to release captured CO2 | 1,000 |
|
Chemical solvent-based system using potassium hydroxide | 365 |
|
Electrostatic absorption on a porous plastic bead with regeneration using a humidity swing process | n/a |
Mineral Carbonation | A process that involves rapid mineralization of CO2 at the Earth’s surface | |
|
Formation of mineral carbonates through a reaction of mineral cations with atmospheric CO2 resulting in a permanent form of CO2 storage | 10,000,000 |
|
Bicarbonate (HCO3-) formation from calcium- and/or magnesium-containing minerals | n/a |
a See http://globalthermostat.com/a-unique-capture-process.
b Peter Eisenberger, Global Thermostat.
c Jan Wurzbacher, Climeworks.
d Geoff Holmes, Carbon Engineering.
e Ishimoto, Y., et al. 2017. Putting Costs of Direct Air Capture in Context. Forum for Climate Engineering Assessment Working Paper Series. FCEA Working Paper Series: 002.
f Greg Dipple, the University of British Columbia.
g Phil Renforth, Cardiff University.
OVERVIEW OF DIRECT AIR CAPTURE APPROACHES
Manufactured DAC
DAC technologies capture and concentrate CO2 from ambient air. A description of various approaches to DAC are outlined in Table 1. DAC is a proven technology used in submarines, spacecraft, and in liquefaction of air, said Klaus Lackner of Arizona State University. In DAC systems, chemical sorbents are used to remove CO2 as ambient air flows over an air contactor. After CO2 is captured, the material used for separation requires regeneration, resulting in the production of a near pure stream of CO2. This CO2 can then be utilized or sequestered.2,3 Jennifer Wilcox of the Colorado School of Mines and Chris Jones of the Georgia Institute of Technology explained how manufactured DAC involves two generic approaches: one being a solvent-based method and another being solid-sorbent-based. In the solvent-based approach, a strong alkaline solution is used to capture CO2 from the air in a simple acid-base reaction that results in the formation of a stable carbonate, which then has to be heated in an oxy-fired kiln to release high-purity CO2 and lime to be recycled throughout the process, as described by Geoff Holmes, Carbon Engineering. In a solid-sorbent-based DAC process, CO2 from the air binds to a sorbent using a gas-solid contactor. An approach based on both heat and vacuum is used to desorb the CO2 from the sorbent, producing a concentrated stream of CO2. This system is subsequently cooled to begin the cycle again.
___________________
2 Ibid.
3 Mazzotti, M., R. Baciocchi, M. J. Desmond, and R. H. Socolow. 2013. Direct air capture of CO2 with chemicals: optimization of a two-loop hydroxide carbonate system using a countercurrent air-liquid contactor. Climatic change 118(1): 119-135.
CCS
Carbon capture and storage (CCS) is the process of capturing CO2 from a concentrated gas stream and storing it deep below ground. The DAC process is similar to CCS in regard to the chemicals used to capture CO2, although unlike direct air capture, CCS activities have been practiced for several decades, explained Ron Munson from the Global CCS Institute. CCS of anthropogenic CO2 for enhanced oil recovery (EOR) has been in operation since the 1970s; for example, the Sleipner project in Norway has been in operation for more than 20 years to date, capturing about 800,000 tonnes of CO2 per year and storing it in a saline aquifer. Another factor that differentiates CCS from DAC, said Munson, is scale. A large-scale integrated CCS facility is defined by the Global CCS Institute as involving the capture, transport, and storage of CO2 at a scale of at least 400,000 tonnes of CO2 annually, whereas current DAC facilities are in the hundreds to thousands of tonnes per year. Consequently, one of the advantages of DAC over conventional CCS is the ability for distributed deployment, which can be used as a mechanism for scale-up moving forward.
CCS tends to be applied to industrial and electricity generation sectors with high concentration of CO2 in the gas streams. As a result, 15 out of 17 operating facilities worldwide capture CO2 from three major types of industries: syngas production, natural gas processing, and ammonia production. Since the concentration of CO2 in ambient air is around 0.04%, compared to 15% and 5% for coal and natural gas power plant emissions, respectively, DAC is thermodynamically more challenging than CCS, and has to deploy novel engineering designs.
Mineral Carbonation
Mineral carbonation refers to the process of chemical weathering, where cations from minerals react with atmospheric CO2 to form mineral carbonates—a permanent form of CO2 storage. One method of mineral carbonation involves extracting cations of magnesium from mine tailings. Mineral carbonation can also occur through the formation of bicarbonates (HCO3-) from calcium- and/or magnesium-containing minerals. Enhanced weathering, where minerals are spread onto a land surface or coastline, is one method for this approach.
CROSS-CUTTING CONSIDERATIONS FOR DIRECT AIR CAPTURE
Impacts
Water and land requirements for DAC are variable. Geoff Holmes indicated that for the Carbon Engineering process, an average of 4–4.5 tonnes of water are evaporated per tonne of CO2 captured, whereas the Climeworks technology captures water from the air at a rate of 1–2 tonnes of water co-adsorped from the air per tonne of CO2. In terms of land use impacts, Holmes explained that a facility that captures a million tonnes per year would require 2 km2 of land. A facility that is capable of capturing a gigatonne of CO2 per year would require approximately 7,000 km2. Holmes emphasized that these numbers are for reference only in order to understand how much land is required to do DAC on a large scale. Other issues, such as capital and material requirements, environmental risks, and aerosol emissions, are very small and do not serve as limiting factors for large scale deployment and demonstration.
Costs
The primary limitation to wide implementation of DAC technology is cost, stated Lackner, as did other webinar speakers. He noted that given the comparatively low prices per tonne of CO2 for flue gas scrubbing versus those for early DAC implementation, air capture technology currently remains unable to deploy commercially absent specific policy measures. A wide range of potential costs of manufactured DAC were discussed during the webinar and at the workshop. Cost estimates ranged from $100–$600 per tonne of CO2, spanning the range of current costs of DAC technology at the pilot scale to projected costs of implementation assuming various trajectories for scaling up.
Lackner explained that the concentration of CO2 in the air is 300 times more dilute than in the flue gas of a coal plant. Thus, DAC technology has to overcome Sherwood’s Rule, which states that the cost of separation scales linearly with dilution.4 In addition to the cost of separation, other costs include the regeneration of the sorbent and the energy requirements for this step. Improvements in sorbent performance and longevity, therefore, could reduce costs. Heat recovery is another important aspect of the cost of DAC technology.
Despite these challenges, Lackner said that the cost of DAC can be driven down. Sherwood’s Rule can be overcome if air contact with the sorbent does not dominate the cost of DAC. This can be achieved through passive, wind-
___________________
4 Sherwood, T. K. 1959. Mass Transfer Between Phases. Priestley Lecture Series. State College, PA: Pennsylvania State University.
driven flow designs or by using very small pressure drops in the ducts of the collectors. If the high cost of this first step is avoided, the cost of sorbent regeneration is only weakly dependent on the original concentration of CO2. In addition, Lackner stated that based on prior precedents from mass manufacturing, technology learning curves have followed cost reductions of 15–20% for every doubling in output. David Keith from Harvard University contended that many factors are involved in predicting the long-term future of early-stage technologies, including economies of scale, changes in factor prices (the price of the means of production), and exogenous technological learning driven by spillovers from other sectors. Even if the cost of a specific technology is well understood from a techno-economic analysis, this analysis cannot be generalized to apply to DAC technology overall. Actual price estimates require working with construction companies and vendors of individual technologies; price estimates also require pilot demonstrations and their interaction at scale. Keith noted that there is, however, some evidence that modular technologies—in a physical and business sense—have faster technology learning rates. For example, the cost of the technology employed by Global Thermostat, which can be constructed in units, has the potential to lower the cost of DAC because it can be mass manufactured.
Other factors
Jan Wurzbacher from Climeworks believes that there are no significant technical limitations to deployment of DAC technology; instead, he identified political and economic limitations as greater challenges. Among the most significant, he listed carbon pricing policies, supportive market policies for materials and fuels made from CO2, uncertainty in the availability of base-load renewable energy, and a lack of understanding and support of DAC technology from the general public. Life cycle analysis (LCA) studies, which may be especially useful in validating the technology as a viable CO2 removal approach, are key solutions to addressing a number of these challenges. In terms of social and political challenges, Alina Chanaewa, Skytree, noted that the long-term future of DAC technology will depend on political priorities, which determine the amount of funding and subsidies that are available for climate change abatement. In addition, said Chanaewa, efforts should be made to improve public perception of CO2 from being considered a hazard and a cause of climate change to being regarded as a valuable feedstock for production of materials and fuels. Chanaewa also emphasized the importance of performing life cycle analyses to understand the implications of net negative emissions. However, according to Eric Ping from Global Thermostat, a key criterion for accelerating DAC proliferation is adapting LCA accounting practices that differentiate between avoided carbon (reduction of carbon from a positive emission stream) and negative carbon (direct removal of carbon from the atmosphere). Current users of CO2 gain no substantial benefit from switching to DAC (a negative carbon emission technology) from existing sources (e.g. ammonia, ethylene production facilities) under current LCA practices. Chanaewa noted further that economic viability of DAC technology will also depend on the price of oil since it regulates the demand for alternatives—low oil prices will make it challenging for the products made out of captured CO2 to compete in consumer markets.
Overall, the DAC industry needs more validation and early commercial projects, which tend to have higher risk profiles than well-established industries, and therefore do not attract traditional financial investors and funds, said Holmes. Since this is a capital-intensive technology, he explained, favorable policies and regulations are needed to help generate the revenues required to make DAC and sequestration commercially viable. Moreover, Wurzbacher noted that field deployment of plants with extended operation periods is necessary to achieve performance optimization of practical DAC processes. Munson further suggested that one of the important components for future development of DAC is a well-defined plan for outreach and education of stakeholders and policy makers to garner a constituency to help support ongoing research.
COMMERCIAL-SCALE APPROACHES TO DIRECT AIR CAPTURE
Direct air capture is being approached using varying processes and for varying end uses by a number of companies. Global Thermostat’s modular technology was described in more detail by Peter Eisenberger from Columbia University. The commercial modules of Global Thermostat have the capacity to capture up to 50,000 tonnes of CO2 per year and can be further scaled up if necessary. In the first step of this particular technology, the air flows parallel to the high surface area of monolith contactors at the speed of 5 m/sec and at the cost of $5/tonne. The parallel surface of the contactors allows for maximizing the contact area for the diffusion of CO2 onto the active material of the sorbent, minimizing the pressure drop. The sorbent, embedded in the walls of the contactor, consists of amines, which have been demonstrated to be very effective at capturing CO2 from the air at 400 ppm. The use of a combination of temperature swing and steam as sweep gas and heat transfer fluid allows for regeneration of the sorbent at 95°C. By recycling heat in a heat transfer process, Global Thermostat has achieved a 50% reduction in thermal requirements for their process.

SOURCE: Jan Wurzbacher
The world’s first commercial plant to capture CO2 from the air opened in Switzerland in 2017 (see Figure 1). Wurzbacher stated that the plant is currently capturing 1,000 tonnes per year. He estimated that the total technically feasible commercial deployment of their technology can reach 10,000,000 tonnes of captured CO2 per year by 2025. Wurzbacher also explored the potential cost and energy requirements for scaling up DAC to remove 300 million tonnes of CO2 per year. He estimated that $100–$300 billion in one-time investments would be required to reach that capacity for captured CO2. Wurzbacher compared that to global investments in renewable energy technology, which totaled $240 billion in 2016. In terms of energy consumption, capturing 300 million tonnes of CO2 would require between 300–600 TWh per year in heat and electricity. This can be met relatively easily by the global renewable energy generation sector, which produces approximately 30,000 TWh of heat and electricity annually.
Climeworks is targeting two sequestration approaches for their CO2 air capture technology: production of renewable fuels or chemicals, and underground sequestration. The latter application is currently done on a small scale in Iceland using geothermally-produced electricity. The CO2 extracted from the air is mixed with water in the CarbFix process, which was developed by Reykjavik Energy and their collaborators. This water-CO2 mixture is injected into 1,000-to-2,000-meter–deep underground basaltic rock formations, which are one of the most abundant rock formations in that area. Once injected, CO2 reacts with basaltic rocks and within about two years forms a solid, which is rapid for a geologic reservoir. High reactivity of the basaltic rock, dissolution of CO2 prior to or during injection, and geothermal properties of the ground increase the speed of the carbon mineralization reaction. The plant is contributing around 100 kilograms of negative CO2 emissions every day. The Climeworks technology process also features the co-adsorption of water and carbon dioxide, an important characteristic for cycle performance and energy consumption as it generally increases both the energy demand of the process but also the purity of the CO2 stream.
A pilot scale DAC plant has been in operation by Carbon Engineering since 2015. Geoff Holmes explained that Carbon Engineering’s air capture technology is based on established processing industry practices, where the system uses a strong alkaline scrubber coupled with multistage regeneration technology to deliver a continuous stream of pure CO2. Primary inputs to operate the facility are energy and water (agricultural- or industrial-grade water can be used in the process). During the process, the water used is evaporated as CO2 is captured—therefore, the system strongly depends on ambient humidity. Between 4–4.5 tonnes of water are evaporated per tonne of CO2 captured. The energy demand to operate the plant is met with a mixture of renewable electricity and natural gas. CO2 emissions produced from natural gas are also captured along with CO2 from air. Carbon Engineering’s pilot plant currently captures 1 tonne of CO2 per day. The estimated capacity of their technology, according to Holmes, is 1 million tonnes of captured CO2 per year. Holmes provided further details on the carbon balance calculations for Carbon Engineering’s natural gas–powered system, taking into account upstream operating and downstream fugitive CO2 emissions, as well as annualized one-time emissions for building and decommissioning the plant. The results show that for each tonne of captured CO2, 0.1 tonnes of CO2 are emitted in the process of capturing and storing, resulting in a net sequestration of 0.9 tonnes. These numbers agree with LCA calculations performed by Climeworks in collaboration with industrial partners and the University of Stuttgart, as explained by Jan Wurzbacher.5 The site emissions of the Climeworks facility are less than 10% of the captured CO2.
Moving forward, Holmes outlined short-term and long-term plans for the Carbon Engineering technology. In the short-term, the company is envisioning to pilot test a facility capable of thermocatalytically coupling CO2, captured by their DAC process, with H2, produced from water electrolysis using renewable electricity, to make synthetic fuels for the transportation sector. The long-term goal is to scale up the air capture operation to 250,000 tonnes of CO2 per year, and, coupled with air-to-fuels technology, produce 2,000 barrels of fuel per day.
___________________
5 Lozanovski, A., C. Gebald, C. P. Brandstetter, J. P. Lindner, and S. Albrecht. 2015. Direct Air Capture of CO2. Presented at 8th Biennial Conference of the International Society for Industrial Ecology, University of Surrey, Guildford, United Kingdom, July 7-10, 2015.
SCALING OUT AND UP OF CCS, CHALLENGES AND LESSONS LEARNED
Despite the fact that CCS has been around since the 1970s, Roger Aines from Lawrence Livermore National Laboratory cited two major reasons for why it has not been successful. First is that the industry attempted to implement CCS at full scale immediately instead of going through the cycles of learning, optimization, and gradual scale-up. Second is the absence of monetary value in carbon-free electricity from coal-fired and gas-fired power plants. Nonetheless, Aines is optimistic about the future of direct air capture technology. The passage of the Low Carbon Fuel Standard (LCFS) in California provides an opportunity for investors to monetize negative carbon emissions by receiving credits for employing DAC to remove CO2 from the atmosphere, followed by subsequent geologic sequestration.
In order to remain competitive, Ron Munson stated that a future R&D program for both CCS and DACS facilities would aim to reduce capital and operating costs. Although DAC technology has a lower cost-per-installation compared to CCS and has the opportunity to offset costs with the production of revenue-generating products, capital and operating costs are substantial for both technologies. Munson suggested that market analysis should be a part of an R&D portfolio for DAC, where targets and metrics are structured based on market definitions as well as future projections, in addition to opportunities in using modular approaches to reduce costs for scaling up. Aines suggested that non-cost issues, such as confidence in accountability and monitoring of LCFS-like systems, access to co-benefits (such as reduced air pollution), and access to new products and new businesses may be more important to DAC and CCS investors. The scale-up of novel processes from a business perspective was explored by Clay Sutton and Tim Barckholtz from ExxonMobil. They also outlined areas of opportunity for DAC technology. Sutton explained that a key component for the DACS systems is integration with low carbon energy sources to provide a continuous power source at the three stages of the DACS process: capture, separation, and compression. At the CO2 capture stage, low pressure drop contactor designs are an important focus area. At the separation step, there are opportunities to increase sorption capacity, reduce regeneration energy input, and increase sorbent lifetimes. For compression, Sutton and Barckholtz stated that research emphasis in this area should focus on the sorbent designs that reduce impurities going into the compressor feed. When thinking about system scale-up, early stage conceptual design is critical and should include the entire system, including supporting facilities, energy sources, integration with sequestration infrastructure, siting, and scale of the equipment, Sutton explained. Compared to earlier remarks regarding the ability of distributed deployment as one of the advantages of DAC, these considerations, in practice, would limit the potential for distributed deployment. Sutton also highlighted the opportunity for modularity to help the cost structure of DAC in scaling up infrastructure.
ADSORBENTS FOR DAC
Novel process designs and system-level considerations for adsorption-based DAC processes were discussed by Matthew Realff from the Georgia Institute of Technology. There are several important elements to consider for such processes. A key challenge in adsorption-based processes is the adsorbent itself, as well as the process of designing adsorbents that are active, stable, resistant to oxygen, and resistant to water degradation. Furthermore, understanding the physical properties of adsorbents at a fundamental level is critical to understanding performance of these kinds of DAC systems. Another consideration is overall operational scheme. When looking at the overall cost and energy components for this type of process, stated Realff, several assumptions have to be made regarding the bulk production cost of the adsorbent, the lifetime of the adsorbent (presumed to be 1–3 years), and electrical and thermal energy use. The results of a cost analysis for two specific metal organic framework (MOF) adsorbents show that adsorbent capital expenditure (CAPEX) is the largest component of the system. Adsorbent lifetime and degradation dynamics are not well understood in real operating conditions; therefore, there is a clear need for understanding how these adsorbents behave in real systems and the extent of their real lifetime, according to Realff. In terms of the energy requirement per tonne of captured CO2, Realff explained that his analysis indicates a mid-range of between 500–1,000 kWh for electricity and 1,000–1,500 kWh for thermal energy. Since the energy demands of a DAC process are both electrical and thermal, Realff speculated that renewable electricity is not necessarily the most efficient source of energy. Realff suggested that in order to accelerate technology optimization and increase the rate of deployment of adsorption-based DAC, it is important to identify and promote applications at different points along the technology adoption curve.
SYSTEMS LEVEL CONSIDERATIONS AMONG NEGATIVE EMISSIONS TECHNOLOGIES
Niall Mac Dowell from Imperial College London discussed system-level considerations of DAC in the context of the whole energy system with specific attention to how negative emissions technologies (NETs) might interact and
compete with one another. Mac Dowell first compared the mitigation potential of direct air capture and sequestration (DACS) versus carbon capture and utilization (CCU) technologies, focusing specifically on utilization to fuels. Mac Dowell stated that his analysis shows that for the potassium hydroxide-based process, DACS provides superior mitigation service compared to CCU in economic and energy terms. For example, curtailed renewable energy, needed to capture and hydrogenate CO2 to produce liquid fuels in the CCU process, would not be available, or would not be available for free, while waste heat, which is needed for DACS processes, would be available at significant levels.
Mac Dowell also addressed how NETs, specifically Bioenergy with Carbon Capture and Storage (BECCS) and DACS, might affect the energy system overall from an economic and energy perspective, assuming a zero-carbon emission target by 2050. In this context, BECCS is a more preferable option to DACS, according to Mac Dowell. BECCS is cheaper, it generates electricity, and provides a similar carbon emission offsetting service as DACS.5 To be attractive relative to BECCS, DACS needs to be significantly cheaper in both capital and operational expenditure terms, observed Mac Dowell. The key advantage of DAC over BECCS is that it removes CO2 from the atmosphere from day one. Depending on the circumstances of the BECCS system, net reduction of CO2 in the atmosphere can take quite some time, if at all, noted Mac Dowell.
Ajay Mehta from Shell had a broader analysis of how DAC fits into the macro picture of the energy system in an emerging net-zero emissions world. He agreed that in order to reach the net-zero emissions target by mid-century, carbon capture and storage (CCS) has to be implemented.6,7 However, CCS would need to reach a scale on the order of 13 gigatonnes of CO2 per year, accompanied with a decrease in fossil fuel consumption by 34%.8 Considering that CCS is currently implemented a scale on the order of 35 to 40 million tonnes per year globally, the question remains as to whether CCS will reach the necessary scale. According to Mehta, Shell is aiming to utilize a DAC to fuels approach by harnessing low-cost solar energy in combination with novel technologies for energy storage and conversion. Specifically, by using low-cost electricity from offshore wind or photovoltaic (PV) systems, Shell is looking to generate hydrogen and capture CO2 for production of synthetic fuels. Mehta offered some suggestions for how to promote a path from DAC to fuels. From the technical perspective, some specific areas of R&D include support for CO2 reduction catalysis methodologies and approaches, improvement in methods for generating green H2, and improvement in multi-scale integrated modeling to steer the design of experiments, testing, and optimization of systems. He noted that regulatory supports to promote market entrance of early-stage CO2 utilization technologies, as well as support for adjacent low carbon technologies such as low carbon energy sources, would be useful. To conclude, Mehta pointed out that standardized LCA methodologies, as well as carbon pricing policies, would be beneficial.
MINERAL CARBONATION APPROACHES
In mineral carbonation, cations from minerals react with atmospheric CO2, forming mineral carbonates and resulting in a permanent form of CO2 storage. Greg Dipple from the University of British Columbia discussed mineral carbonation in mine tailings (see Figure 2). He stated that the process is currently occurring naturally on a scale of 40 kilotonnes of CO2 per year. If mining is specifically performed for the purpose of carbon sequestration, it is feasible to achieve a scale on the order of hundreds of megatonnes to gigatonnes per year. Currently, the global CO2 capture capacity of mines that extract commodities is around 200 million tonnes of CO2 per year.
The carbon footprint of mining operations is significant. Furthermore, if mining is performed for the purposes of air capture, land impacts have to be considered as well. For example, mineral carbonation on the scale of 1 million tonnes of CO2 per year would require 83 km2 of land for the purpose of mine tailing storage. Compared to estimated land requirements for DAC of 2 km2, as presented by Geoff Holmes, this is a significant demand on land resources for the same amount of CO2.
Due to environmental implications and resource availability, it is important to consider the rates of mineralization as well as the amount of cations that are available for the process of mineralization. According to Dipple, tenfold acceleration of the rate of carbon mineralization offsets mine emissions, and hundredfold acceleration would achieve mineralization of 1 million tonnes of CO2 per year. Lab and field evidence indicate that carbonation rates are limited by CO2 supply, but if the assumption is that the CO2 supply limit can be easily overcome, the rate of the reaction becomes
___________________
6 Fajardy, M. and N. Mac Dowell. Can BECCS deliver sustainable and resource efficient negative emissions? 2017. Energy & Environmental Sciences 10:1389-1426.
7 Fajardy, M. and N. Mac Dowell. 2018. The energy return on investment of BECCS: Is BECCS a threat to energy security? Energy & Environmental Sciences, Accepted.
8 Energy Transitions Commission. 2017. Better Energy, Greater Prosperity Achievable Pathways to Low-Carbon Energy Systems.
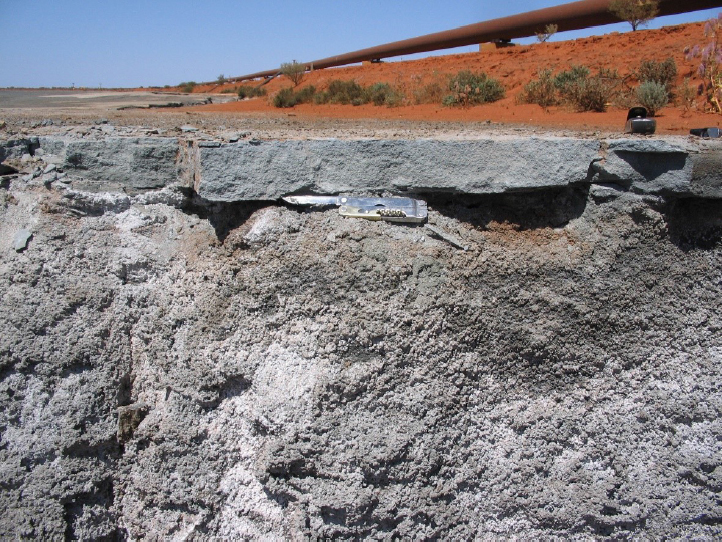
LOCATION: Mt. Keith Nickel Mine, WA, Australia.
SOURCE: Greg Dipple
limited by the rate at which cations of magnesium can be extracted from mine tailings. Easily extractable (labile) magnesium is present in trace minerals, such as brucite. Additionally, some other minerals, such as serpentine, can also release substantial amounts of Mg2+ due to their high surface area. Labile magnesium is equated to cheap carbon sequestration at a relatively low price per tonne.9 However, the content of labile Mg2+ in geologic deposits is inherently variable, and as a result, the cost of sequestered CO2 will vary depending on the weight percent (wt%) of labile magnesium in the ore: the cost of CO2 mineralization is much higher at 2 wt% of labile magnesium than at 9 wt%.
There are a number of challenges and opportunities for carbonation of CO2 in mine tailings. Dipple indicated that some of the challenges for mineralization are similar to the challenges of DAC technology described earlier, such as enhancing the efficiency of capture of CO2 from ambient air and increasing air-water circulation. Dipple also suggested that the following areas more specific to CO2 mineralization can benefit from further R&D efforts: tools for labile cations, mineral pre-treatment, land-use verification protocols for tailings storage, and other ways of delivering carbon, such as solution mining and sub-surface mineralization. A regulatory framework to allow for experimentation on the pilot scale is also needed, he noted.
Another approach for mineral carbonation of CO2 is the formation of bicarbonates (HCO3-) from calcium- and/or magnesium-containing minerals. Phil Renforth, Cardiff University, explained that the benefit of this approach is the ratio of carbon to cation, which is 2:1, rather than 1:1 as it is in the case of carbonates (CO32-). This is important if the availability of labile magnesium is a bottleneck. In addition, this approach allows for the use of carbonate minerals to facilitate carbon sequestration. However, bicarbonates are less stable than carbonates, and the former will eventually precipitate as carbonates, reversing the carbon storage. Therefore, in order to inhibit this reaction, bicarbonates have to be stored in the ocean; although bicarbonate concentration in the oceans is quite large, the residency time of HCO3- is on a scale on the order of 1 million years. However, adding bicarbonates to the oceans might potentially affect ocean dynamics and CO2 sequestration times, although resonance time is still likely to remain substantial, noted Renforth. Adding bicarbonates to the oceans would lead to elevated ocean alkalinity compared to current levels. The environmental impact to the ecosystems is under debate. Renforth suggested that if this approach is used as a mitigation strategy, the carbonate saturation state would likely return to pre-industrial levels, and therefore be benign from an environmental perspective. However, some studies suggest that if the addition of excess bicarbonates is concentrated in one or two locations rather than distributed, the environmental impact is severe.10 There are several ways for
___________________
9 Dipple, G. M., I. Power, K. Carroll, and B. De Baere. 2017. Pathways to Accelerated Carbon Mineralization in Mine Tailings. In CO2 Summit III: Pathways To Carbon Capture, Utilization, And Storage Deployment. Eds. ECI Symposium Series. http://dc.engconfintl.org/co2_summit3/16.
10 Ilyina, T., D. Wolf-Gladrow, G. Munhoven, and C. Heinze. 2013.Assessing the Potential of Calcium-Based Artificial Ocean Alkalinization to Mitigate Rising Atmospheric CO2 and Ocean Acidification. Geophysical Research Letters 40:5909–5914.
the addition of bicarbonates to the ocean to be accomplished. One approach is enhanced weathering, where minerals are spread onto the land surface or coastline. Other approaches involve the application of bicarbonate minerals to the upwelling regions of the ocean, ocean liming, and electrochemical weathering. The costs of sequestering CO2 via enhanced weathering can vary over a large range, from tens of dollars to over hundreds of dollars per tonne of CO2, with energy requirements ranging between 220 kWh and 3,500 kWh per tonne of CO2 for basic and ultrabasic rocks.11 The reason for such variation, explained Renforth, is due to variation in large scale versus lab reaction kinetics; catchment-scale kinetics seem to be far slower than the lab-based ones. If faster reaction rates occur, less comminution of rocks is needed. In terms of scale, Renforth suggested that sequestration of 1–2 gigatonnes of CO2 may be feasible using this approach. Renforth noted that despite high energy and economic costs, enhanced weathering may have added co-benefits, including soil fertilization (in the case of basalt), crop resilience to pests, and recovery of soil erosion losses. Nevertheless, enhanced weathering still requires comprehensive monitoring and accounting due to several potential risks, such as fertilizing terrestrial and coastal ecosystems and metal accumulation. Furthermore, comminution and distribution of rock leads to generation of dust on the production and application site, carrying exposure health risks.12
___________________
11 Renforth, P. 2012. The Potential of Enhanced Weathering in the UK. International Journal of Greenhouse Gas Control 10:229–243.
12 Taylor, L. L., J. Quirk, R. M. S. Thorley, P. A. Kharecha, J. Hansen, A. Ridgwell, M. R. Lomas, S. A. Banwart, and D. J. Beerling. 2016. Enhanced Weathering Strategies for Stabilizing Climate and Averting Ocean Acidification. Nature Climate Change 6:402-406.
DISCLAIMER: This Proceedings of a Workshop—in Brief was prepared by Anna Sberegaeva and Yasmin Romitti, National Academies of Sciences, Engineering, and Medicine as a factual summary of what occurred at the meeting. The statements made are those of the rapporteur or individual meeting participants and do not necessarily represent the views of all meeting participants; the planning committee; or The National Academies of Sciences, Engineering, and Medicine.
REVIEWERS: To ensure that it meets institutional standards for quality and objectivity, this Proceedings of a Workshop—in Brief was reviewed by Mark Saeys, Ghent University, Julio Friedmann, Carbon Wrangler, LLC, Eric Shen, ExxonMobil, Dane Boysen, Modular Chemical Inc., and Niall Mac Dowell, Imperial College London.
Committee for Developing a Research Agenda for Carbon Dioxide Removal and Reliable Sequestration: Stephen Pacala (NAS, Chair), Princeton University, Mahdi Al-Kaisi, Iowa State University, Mark Barteau (NAE), Texas A&M University, Erica Belmont, University of Wyoming, Sally Benson, Stanford University, Richard Birdsey, Woods Hole Research Center, Dane Boysen, Modular Chemical Inc., Riley Duren, Jet Propulsion Laboratory, Charles Hopkinson, University of Georgia, Christopher Jones, Georgia Institute of Technology, Peter Kelemen (NAS), Columbia University, Annie Levasseur, École de Technologie Supérieure, Keith Paustian, Colorado State University, Jianwu (Jim) Tang, Marine Biological Laboratory, Tiffany Troxler, Florida International University, Michael Wara, Stanford Law School, Jennifer Wilcox, Colorado School of Mines.
SPONSORS: This Workshop was supported by the U.S. Department of Energy, the National Oceanic and Atmospheric Administration, the Environmental Protection Agency, the United States Geological Survey, the V. Kann Rasmussen Foundation, and Incite Labs, with support from the National Academy of Sciences’ Arthur L. Day Fund.
For additional information regarding the Workshop, visit http://nas-sites.org/dels/studies/cdr.
Suggested citation: National Academies of Sciences, Engineering, and Medicine. 2018. Direct Air Capture and Mineral Carbonation Approaches for Carbon Dioxide Removal and Reliable Sequestration: Proceedings of a Workshop—in Brief. Washington, DC: The National Academies Press. doi: https://doi.org/10.17226/25132.
Division on Earth and Life Studies
Copyright 2018 by the National Academy of Sciences. All rights reserved.