3
Factors Influencing Smallpox Readiness and Response
“There are two opportunities right now for smallpox medical countermeasures research and development—the opportunity to innovate in manufacturing with a direct impact on what we can stockpile in the future, and the opportunity to better understand the safety and efficacy of these products in the context of mpox response.”
Matthew Hepburn, in presentation to the committee December 15, 2023
Federal smallpox planning in the post-eradication era has been characterized by strategies to reduce the risk that an outbreak would pose, primarily through containment using medical countermeasures (MCMs). Risk reduction can be approached by diminishing the threat itself, the vulnerability to the threat, or the consequences of the threat once it has materialized. The U.S. government has heavily invested in the stockpiling of smallpox vaccine, an MCM risk reduction approach intended to reduce population vulnerability after an outbreak has been detected. The effectiveness of smallpox MCMs (whether diagnostics, vaccines, or therapeutics) as agents of risk reduction rests on numerous factors, from a working knowledge of smallpox biology and epidemiology to the risks and benefits of emerging science and technology to myriad operational planning decisions. Hence, the clinical efficacy of MCMs alone is insufficient to predict its risk-reduction efficacy in an actual outbreak, as MCM distribution and uptake depend on many fiscal, operational, social, and political considerations. These latter considerations, while central, fall outside the scope of this committee, which has focused its efforts on the countermeasures themselves. Similarly,
sociopolitical, lab safety, and other strategies to reduce the risk of a smallpox outbreak taking place are also outside the scope. Still, the findings or conclusions related to MCMs in this report should be viewed holistically and as operating within these other considerations.
EVOLVING BIOTHREAT LANDSCAPE
As noted by the World Health Organization (WHO) Advisory Committee on Variola Virus Research (ACVVR) in 2023, the context for smallpox readiness has changed due to a dramatically different risk landscape, including changes in population (e.g., demographic, physiological, and behavioral/risk perception), since eradication and especially so in recent years:
Salient elements included the waning of immunity against smallpox in the global population, the advent of the HIV/AIDS pandemic and greater prevalence of other immunosuppressive conditions, the continuing advance of synthetic biology and biotechnology making de novo synthesis of viral pathogens possible, the continuing evolution of orthopoxviruses including genetic features suggesting adaptation to more efficient human-to-human transmission, and the observation that currently available countermeasures may not be sufficient to contain outbreaks in the face of a more transmissible and pathogenic orthopoxvirus (WHO, 2024a).
Routine smallpox vaccination ended in 1972 in the United States and globally by 1984. While some studies suggest that protection from vaccination during eradication efforts may be long-lasting, the extent to which this is the case is not well understood, and the durability of vaccination against clinical smallpox for all current vaccines is unknown (Hammarlund et al., 2003; Taub et al., 2008). While there was a brief attempt to vaccinate a larger fraction of the potentially at-risk civilian population against smallpox in the months leading up to the war in Iraq, this effort largely failed for sociopolitical reasons (IOM, 2005). Presently, only individuals with occupational health risk to smallpox and orthopoxviruses receive inoculation, such as laboratory and health care personnel working with orthopoxviruses and around 1.4 million current and former U.S. military members who could potentially provide essential services in the event of a smallpox outbreak (Decker, et al., 2021; Grabenstein and Winkenwerder, 2003; Petersen et al., 2016). There are questions about duration of protection among non-military U.S. personnel vaccinated since 1972 due to improper application of the scarification technique used for vaccine administration (Kennedy and Gregory, 2023). Individuals who have been vaccinated against mpox using modified vaccinia Ankara-Bavarian Nordic (MVA-BN) are expected to have protection against smallpox (though some scientists posit that the decline of immunity to variola
may be a contributing factor in the rising frequency of mpox outbreaks (Durski et al., 2018; Van Dijck et al., 2023)). Therefore, the population of individuals who have been vaccinated with an approved smallpox vaccine since the end of routine vaccination is a very small proportion of the U.S. population. Furthermore, the number of people at risk of serious adverse reaction to replicating smallpox vaccine has grown since eradication (e.g., there are more people now living with HIV and taking immunosuppressant drugs) with one study suggesting that approximately 15 percent of the U.S. population is contraindicated to replicating smallpox vaccine, rising to about 38 percent if their household contacts are included (Carlin et al., 2017; Parrino and Graham, 2006).
It has long been known that accidental or deliberate outbreaks remain possible due to existing and allowed viral collections, and even natural outbreaks are at least theoretically possible if there is viable virus in, for example, thawing permafrost, but recent advances in synthetic biology technology now mean that the variola virus or another microbe that could cause smallpox-like illness could be recreated in a laboratory even if all known collections of variola virus were to be verifiably destroyed (Jaschke et al., 2019; Levrier et al., 2023; Noyce et al., 2018; Reardon, 2014, Thi Nhu Thao et al., 2020; WHO, 2015). Furthermore, since routine smallpox vaccination ended in 1972 in the United States and globally in the ensuing years, population-level immunity has waned. Some scientists posit that the decline of immunity to variola may be a contributing factor in the rising frequency of mpox outbreaks (see Box 3-1) (Durski et al., 2018; Van Dijck et al., 2023).
The changing threat landscape is further evidenced by the increasing frequency and scope of orthopoxvirus outbreaks in recent years (Box 3-1). Mpox outbreaks have occurred sporadically in Central and East Africa (clade I) and West Africa (clade II) since the 1970s (WHO, 2023b). However, an increase in cases, coinciding with improved surveillance, was observed in west and central African countries in the last decade, prior to the 2022 multi-country outbreak (Durski, 2018).
As exemplified by the 2022 and 2023 mpox outbreaks, any orthopoxvirus may become more evolutionarily adapted to humans through alterations in characteristics like transmissibility or virulence. A more comprehensive understanding of basic orthopoxvirus biology, virus–host interactions, and interactions among viral proteins and other molecules could support development of safer and more effective vaccines and therapeutics, as described further in this chapter and in Chapter 4.
Comparative Poxvirology and Implications for MCMs
There are twelve species of poxviruses across four poxvirus genera that are known to infect humans—orthopoxvirus, parapoxvirus, yatapoxvirus,
BOX 3-1
Notable Orthopoxvirus Outbreaks (as of February 2024)
Mpox
Clade I monkeypox virus outbreaks occur regularly in Cameroon, Central African Republic, and the Democratic Republic of the Congo and sporadically in others (e.g., Sudan, South Sudan).
In 2003, 71 cases (35 confirmed, 36 suspect and probable cases) of clade II monkeypox virus were reported in six U.S. states (Illinois, Indiana, Kentucky, Missouri, Ohio, and Wisconsin) after contact with infected pet prairie dogs housed near imported small mammals from West Africa (CDC, 2003). This was the first time human mpox was reported outside of Africa.
In 2017, clade II monkeypox virus reemerged in Nigeria after almost four decades of no reported cases (Yinka-Ogunleye et al., 2018).
Since 2022 the clade IIb monkeypox virus has spread globally, affecting many countries without previous mpox cases. Transmission was driven via sexual contact among men who have sex with men.
As of January 28, 2023, 13,357 total mpox cases (714 confirmed cases) and 715 suspected mpox deaths having been reported from 22 out of 26 (85 percent) provinces in the Democratic Republic of Congo (WHO, 2023a). This is the highest number of annual cases ever reported, with new cases in geographic areas that had previously not reported mpox, including Kinshasa, Lualaba, and South Kivu. Clade I monkeypox virus has been confirmed among tested cases.
Alaskapox
The first case of Alaskapox virus infection resulting in hospitalization and death was reported in February 2024 (Hedberg, 2024). Since the first human case was discovered in 2015, six additional cases in humans have been confirmed in the Fairbanks area. The probable animal reservoirs include voles and squirrels. To date, no person-to-person transmission has been documented; domestic animals may play a role in spreading the virus (Alaska Department of Health, 2024).
and molluscipoxvirus (Table 3-1). Variola virus is a member of the orthopoxvirus genus within the Poxviridae family. Poxviruses are large and possess a single linear, double-stranded DNA molecule of 130–375 kilobase pairs, depending on the species, which can encode more than 200 proteins (Burrell et al., 2017). Only two viral species of poxviruses are obligate human pathogens: variola virus (orthopoxvirus genus) and molluscum contagiosum (molluscipoxvirus genus). Vaccinia, the prototype poxvirus and basis of modern smallpox vaccines, is also a member of the orthopoxvirus genus and has occasionally caused disease in humans following vaccination. Cowpox virus and orf virus (genus parapoxvirus) primarily affect livestock but may also infect humans. Many other viral species across the poxvirus
TABLE 3-1 Poxviruses That Infect Humans
Genus | Virus | Reservoir Hosts | Other Infected Hosts | Geographic Distribution |
---|---|---|---|---|
Orthopoxvirus | Alaskapox | ? Voles and squirrels | ? | Alaska |
Cowpox | Bank voles, long-tailed (wood) mice | Cats, rats, cattle, zoo animals | Europe, western Asia | |
Monkeypox* | ? Unknown—likely rodent | Monkeys, zoo animals, prairie dogs | Western, central Africa, worldwide outbreaks | |
Vaccinia | ? | Rabbits, cows, river buffaloes | ? | |
Variola* | Humans | None | Eradicated (formerly worldwide) | |
Parapoxvirus | Bovine papular stomatitis | Cattle | None | Worldwide |
Orf | Sheep, goats | Ruminants | Worldwide | |
Pseudocowpox | Cattle (dairy) | None | Worldwide | |
Seal parapoxvirus | Seals | None | Worldwide | |
Yatapoxvirus | Tanapox virus | ? | None | Eastern and central Africa |
Yabapox | ? Primates | None | Western Africa | |
Molluscipoxvirus | Molluscum contagiosum | Humans | None | Worldwide |
NOTE: Unknown information is indicated with “?”; viruses capable of respiratory routes of human-to-human transmission are indicated with “*”.
SOURCES: Table adapted from Satheshkumar and Damon (2021). Cowpox reservoir hosts from Ninove et al. (2009).
genera affect a wide range of animals, including mammals, fish, birds, and reptiles. These viruses have traditionally been named based on the species of first isolation, and these names may not be reflective of the reservoir species or host range (Moss and Smith, 2021).
As noted in Chapter 1, this report primarily discusses the features of orthopoxviruses and implications for MCMs, due to the greater potential for orthopoxviruses to infect humans. However, the committee recognizes the theoretical potential for spillover of other poxviruses to occur from animal reservoirs and cause disease in humans.
The orthopoxvirus genus comprises many of the mammalian viruses and consists of 13 species, including variola virus—which is unique in its
human-restricted host range—and monkeypox virus, a zoonotic infection that has caused outbreaks in humans for many decades in certain areas of the world (McInnes et al., 2023; Satheshkumar and Damon, 2021). Diversity among variola virus strains is also present across the species and could have implications for MCM efficacy.
Orthopoxvirus Pathogenesis in Humans
Variety across the orthopoxvirus genus, the adaptation of variola virus to a single host (i.e., humans), and its exceptional virulence together imply the presence of a complex set of mechanisms of pathogenesis as well as a complex relationship between host and pathogen which is poorly understood. Knowledge of smallpox pathogenesis and immunity is limited since many of the tools and concepts of modern immunology were not available when smallpox was eradicated. Hence, the understanding of smallpox protection is largely based on epidemiologic studies and analysis of immune response to immunization with vaccinia virus. A lack of detailed understanding of host–pathogen interactions within these viruses makes it impossible to predict the zoonotic potential for viruses in the genus.
Orthopoxvirus pathogenesis mechanisms are also generally poorly understood, including for variola major and minor, monkeypox virus clades I and II, and other orthopoxviruses. Although genome sequences across orthopoxviruses are very similar, the diseases that these pathogens cause may manifest quite differently in terms of morbidity, symptomatology, and death rates. For example, hemorrhagic smallpox is the least common but most virulent of the five manifestations of smallpox disease and resulted in nearly 100 percent mortality. Hemorrhagic disease may have manifested because of deficient host protective responses. Pregnant women were also susceptible. Prior vaccination was not protective, and subsequent infected individuals developed the same spectrum of clinical manifestations as those exposed to a person with ordinary smallpox. There are no studies reported in the open-source literature regarding modification of clinical disease in the setting of a large, deliberate aerosol release of variola virus. Nonetheless, virus particles acquired by the aerosol route for respiratory pathogens penetrate the lower respiratory tract and cause severe symptoms, even in immunocompetent persons (Yezli and Otter, 2011). While patients with hemorrhagic smallpox have displayed high levels of circulating virus, they were not considered unusually infectious; however, failure to recognize smallpox among patients with hemorrhagic clinical presentation sometimes led to the spread of hospital-acquired infections (Bray and Buller, 2004).
Variola major was observed more commonly than variola minor and resulted in an average case fatality of 30 percent (range, 20-40 percent)
during outbreaks in the 20th century, although there was variability even beyond this range (Breman and Henderson, 2002). For variola minor, mortality ranged from 1 to 2 percent (Berche, 2022). Comparatively, mortality from mpox has ranged from 1 to 10 percent depending on the clade and the study, with clade I resulting in higher fatality rates (Gong et al 2022; Jezek and Fenner, 1988). Active surveillance studies in the 1980s revealed a case fatality rate of 9.8 percent among 338 individuals infected with clade I monkeypox virus in the Democratic Republic of the Congo. All deaths were in unvaccinated children (Jezek and Fenner, 1988). In 2023, WHO reported a case fatality rate of 4.8 percent from suspected clade I disease in the Democratic Republic of the Congo (WHO, 2023a). Clade IIa monkeypox virus caused confirmed infections in 37 individuals in the United States in 2003. No mortality was reported (Reynolds et al., 2007). A narrative review of studies regarding the global outbreak of clade IIb monkeypox virus, largely affecting men who report having sex with men, reported a case fatality rate of 0.1 percent (Antinori et al., 2023). Other orthopoxviruses and poxviruses that infect humans, such as cowpox, vaccinia, orf, or molluscum, cause localized disease and minimal morbidity or mortality in immunocompetent people.
Understanding Immune Evasion and Species Tropism
Understanding immune evasion and species tropism allows for a better understanding of viral pathogenesis and might lead to the development of additional therapeutic options. Knowledge of the features of human tropism of variola virus might also produce insights into the potential for “natural” (or unnatural) evolution of a variola-like virus, presenting challenges and opportunities related to dual use research of concern. Understanding host tropism may also aid in the identification of the animal reservoirs of poxviruses, with implication for One Health management approaches.
While poxviruses can bind to and enter a wide range of mammalian cells, their success in replicating depends on a variety of host-related factors, including cell type, cell cycle status, and intracellular signaling events associated with immunity and cellular apoptosis (IOM, 2009b). The study of viral genes that influence host range and the elucidation of their interactions with host proteins and signaling pathways have improved the understanding of tropism. Poxviruses encode many genes that modulate host antiviral responses (Moss and Smith, 2021). These include secreted viral proteins that bind host cytokines, chemokines, and complement proteins as well as intracellular antagonists that block key signaling pathways, thus precluding establishment of an antiviral state, apoptosis, or proinflammatory response (IOM, 2009b). This wide-ranging capacity to antagonize immune responses is likely to be a factor in the exceptional virulence of variola.
A strong affinity for one host species, sometimes referred to as species “tropism,” appears to be generally driven by virus genus- and species-specific genes, as opposed to genes in highly conserved regions of the orthopoxvirus genome (Moss and Smith, 2021). Fully one-third to one-half of poxvirus genes are in the immunoregulation/host interaction category, about which we have only limited understanding (Gubser et al., 2004; Smith et al., 2013). Some exciting advances in recent years have improved the understanding to an extent, such as research on myxoma virus in rabbits exploring the virus’ interaction with host antiviral protein kinase R (Peng et al., 2016) and with SAMD9, which binds the C7L family of proteins that is widely distributed among poxviruses (Liu et al., 2011). An investigation into SAMD9 showed that it is an anticodon nuclease that inhibits codon-specific protein synthesis (Zhang et al., 2023).
Structure and Replication
Orthopoxviruses possess a characteristic brick-shaped form with internal structures resembling a dumbbell with two lateral bodies. This structure is highly conserved among poxviruses, and its distinctiveness allows for the use of electron microscopy (EM) as a diagnostic tool that reveals the presence of a poxvirus. EM use is limited, however, in that it cannot discriminate between poxviruses (except parapoxviruses when using negative-stain EM) (Petersen and Damon, 2015).
EM has nevertheless revealed a wealth of information about viral structure. Virions are individual viral particles produced through the act of viral replication (Moss and Smith, 2021), and virion structure has important implications for antiviral development, especially as it relates to the mechanisms of cell entry, the knowledge of which has largely been informed through studies of vaccinia.
A unique feature of poxviruses is that they replicate solely in the cytoplasm of infected cells, in cytoplasmic “viral factories” where immature virions are produced. These develop into mature virions (MVs) that are fully infectious and which may be released with cell lysis or may become wrapped by trans-Golgi and endosomal cisternae, then transported to the cell periphery where they can fuse with the plasma membrane and become released as infectious enveloped virions (EVs). These EVs then become infectious to other cells, entering these cells by endocytosis or by fusion at the plasma membrane. Once inside a new cell, the virions act quickly and early mRNA synthesis in the cytoplasm can be detected within 20 minutes as the cycle begins anew (Moss and Smith, 2021). Early mRNAs encode many immunoregulatory proteins, intermediate transcription factors, and DNA replication enzymes/factors. This fast action can help facilitate the rapid spread of virus from cell to cell.
Progression through this life cycle involves the action of a significant number of virally encoded products and the participation of host proteins and intracellular structures. The viral proteins that mediate the lifecycle progression represent potential targets for antiviral therapy (Figure 3-1). Viral proteins may play an important role in viral resistance to therapeutics, such as tecovirimat (Chapter 2), which targets a protein that is vital for MV envelopment into EV and required for cell-to-cell spreading. Antiviral agents developed to date work by interrupting viral DNA replication (cidofovir and brincidofovir) or the envelopment process (tecovirimat). Additional antivirals with distinct mechanisms of action—e.g., directly targeting the virion components and many other steps of viral replication within the host—have not yet been explored for smallpox. The intentional development of such antivirals would benefit from high-resolution images of structures and improved knowledge of virion function and poxvirology. Virion structures and compositions, their immunogenicity, and their mechanisms of function are also critical pieces of information for the support of subunit vaccine development.
As described above, viral replication occurs in the cytoplasm (Moss and Smith, 2021). A process called uncoating aids in the release of DNA from the
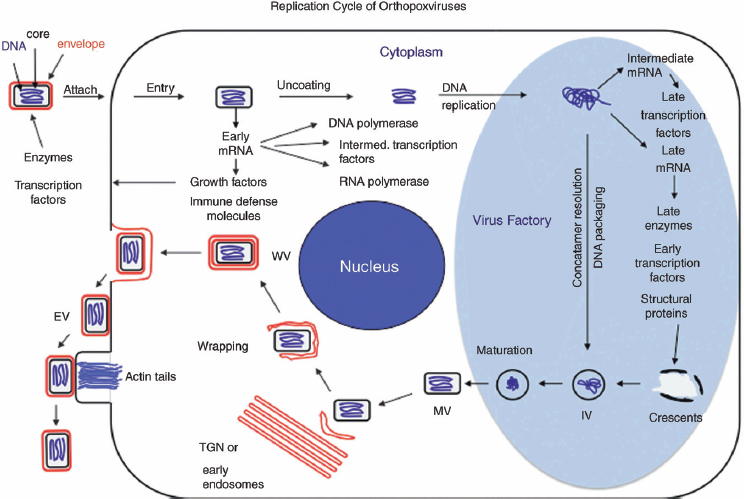
SOURCE: Moss and Smith (2021), Figure 16.6. Reproduced with permission.
virus core—DNA that can then serve as the template for DNA replication. The host’s proteasome inhibitors can prevent uncoating, a mechanism that is not well understood. The newly synthesized viral DNA serves as the template to transcribe intermediate mRNAs, which encode late transcription factors to initiate late transcription. Intermediate and late mRNAs encode structural proteins. These structural proteins and DNA genomes are assembled into viral particles in a complex process that is not well understood (Moss and Smith, 2021).
Each of these replication steps could be targeted for specific antiviral development, but the understanding of many of these steps is limited. For instance, science has not identified the receptors used for poxvirus entry, and it is possible that poxviruses use an unconventional entry mechanism. In addition, there are many outstanding questions regarding DNA replication, transcription, virion assembly, envelope morphogenesis, and egress. These knowledge gaps present barriers to the further development of specific and novel antivirals, to the improvement of vaccines, and to reducing complications associated with vaccines and therapies.
Orthopoxvirus Evolution and Implications for MCM Utility
Orthopoxvirus evolution appears to have been influenced over time by gene loss, fragmentation, and duplication, with subsequent impacts on such features as virus host range and virulence (IOM, 2009b). One analysis identified 49 conserved genes across 21 members of the Poxviridae family, many of which encode key functions such as replication, transcription, virion assembly, or maturation (Upton et al., 2003). These genes are located toward the central region of the genome, in contrast with the more terminal location of genes that are more virus- or host-interaction-specific.
More recent studies during the 2022 mpox outbreak demonstrated previously unappreciated host–viral characteristics, such as viral gene mutations possibly driven by the host antiviral enzyme APOBEC3, which reveal mechanisms of viral adaptation as well as important clues to the evolution of transmissibility (Ndodo et al., 2023; O’Toole et al., 2023). APOBEC3 may mutate DNA through the short-inverted repeats (IRs) in the viral genome, with one study showing that IRs (not the inverted terminal repeats) are indeed a hot spot for mutation (Dobrovolná et al., 2023).
Within genera of poxviruses, the identification of conserved targets or antigenic epitopes among different poxviruses could facilitate the development of broad-spectrum antivirals and vaccines. It may also be possible to look for more broadly cross-reactive poxvirus targets of drugs and vaccines. Genomic similarity and variation among poxviruses, as well as mutation/deletion/addition, will affect the specificity and sensitivity of PCR-based diagnostic assays.
Utility of Orthopoxviruses for Vaccines and Therapeutics
Importantly, some orthopoxviruses have been used as tools against smallpox. Vaccinia virus, an orthopoxvirus that is the basis of currently licensed smallpox vaccines, was cultivated in laboratories over decades for the purposes of vaccinating against smallpox, though the ancestral orthopoxvirus that it originated from is unknown. Vaccinia virus provides benefits to fight against smallpox and other highly pathogenic orthopoxviruses (Jacobs et al., 2009; Torres-Domínguez and McFadden, 2019). Orthopoxviruses have also been studied for their utility as vaccine and oncolytic virotherapeutic agents against various infectious human and animal diseases and cancers (Pastoret and Vanderplasschen, 2003; Perdiguero et al., 2023).
Remaining Gaps
WHO eradication effort was a remarkable success at eliminating smallpox disease from the human population. Though viral collections remain, the public health feat of the disease’s eradication remains an amazing human achievement. The success of that achievement was predicated on the human–host specificity of variola: human-to-human transmission could be successfully interrupted permanently because there was no concern of spillover from another host species and no human chronically infected state.
Yet, considerable gaps remain in understanding variola virus to inform improved MCM development as well as in current understanding of other orthopoxviruses with one or more mammalian reservoir hosts that can infect humans (Moss and Smith, 2021). If speculation that the smallpox virus at one point jumped from animals to human and eventually adapted to be a human-specific pathogen is correct, then other zoonotic poxviruses might follow a similar path of modification to their host tropisms. This represents one possible pathway to renewed outbreaks of smallpox or smallpox-like illness among humans.
Attracting funding for experimental work with smallpox virus to advance more fundamental understandings is challenged by the difficulty of linking such work directly to an “essential” public health need as well as by sociopolitical and ethical concerns about funding dual use research (Damon et al., 2014). The WHO ACVVR has taken a conservative approach to interpreting the World Health Assembly’s resolution on approval of research with variola, leading it to use a relatively restrictive lens when deciding whether to approve work with live virus based on what it perceives constitutes an essential public health need. In particular, “discovery research” with live variola virus (VARV) (i.e., research for its own sake), while potentially interesting and useful, is not consistent with the National Academies’
2009 conclusions, which still suggested that targeted basic science research on variola could support better understanding of pathogenesis and human immune response, with direct implications for improved readiness for smallpox and other orthopoxviruses (IOM, 2009b). The disease ecology of the orthopoxviruses is poorly understood. Susceptible species are not infrequently recognized (see Table 3-1). The impact of where infectious exposure occurs likely impacts disease acquisition and transmission. For example, captive zoo animals and prairie dogs have been shown to be susceptible to mpox infection (Jezek and Fenner, 1988). Prairie dogs efficiently transmitted mpox disease to humans in the United States in 2003 (Reynolds et al., 2007). Zoo animals have also been susceptible to cowpox virus infections (Costa et al., 2023). Box 3-2 reveals a suite of poxvirus knowledge gaps that may be relevant for smallpox MCM research, and which is discussed in further detail in Chapter 4 and specifically Table 4-2.
Conclusions on Contribution of Basic Poxvirus Research to Smallpox Readiness
(3-1) The increasing recognition of orthopoxvirus illnesses in humans merits ongoing research and development of MCMs to detect, prevent, treat, and respond to these diseases. This is of particular importance for mpox that is an ongoing global outbreak and is expected to be a long-term threat. Other emerging orthopoxviruses (e.g., Alaskapox, cowpox, and vaccinia-like viruses) also need to be closely monitored as the population immunity against orthopoxviruses continues to wane.
(3-2) Variola virus-specific research is extremely restricted and is only undertaken when it is necessary and essential for public health. It is not possible to fill knowledge gaps without the study of other orthopoxviruses.
(3-3) Gaps exist in the fundamental understanding of variola virus and non-variola orthopoxvirus biology, pathogenesis, immunity and host interactions, evolution, transmission, and ecology. Basic poxvirus research is beneficial to smallpox MCM development and contributes to readiness against other known and potential novel orthopoxviruses affecting humans. General advances in developing orthopoxviruses as vaccine vectors, gene delivery, and oncolytic virotherapy can have multiple benefits, including enhancing smallpox MCMs.
EVOLVING RESEARCH AND TECHNOLOGY LANDSCAPE
As biotechnologies continue to evolve at a remarkable pace—a pace increasingly accelerated by advancements in artificial intelligence—it is incumbent on decision makers to consider what impacts and opportunities may arise for MCM strategies. Malicious exploitation of such technologies
BOX 3-2
Poxvirus Knowledge Gaps
Genomics
- Detailed knowledge of genomic evolution.
- Mechanisms for genomic DNA editing that lead to quick adaptation in new hosts.
- Adaptation trends in pathogenesis at the genomic level and tools to forecast future outcomes.
Viral Structure and Replication
- Many aspects of viral lifecycles, e.g., receptor(s) and entry mechanisms, DNA replication mechanism, virion assembly, and egress.
- High-resolution imagery of virion structures and structures at various stages of viral replication.
Immune Responses
- Immunological elements that are central to elevating immunity against new infection.
- Immunodominant antigens/epitopes of all human-infectable poxviruses.
- Host and viral elements that determine breakthrough infections.
- Immunoregulation mechanisms of numerous poxvirus immune evasion proteins.
Host–Pathogen Interactions and Mechanism of Host Tropism
- Knowledge of the hundreds of proteins and their functional interactions with viral and host functions that can shape viral transmission, host range, host immunity, virulence, and pathogenicity.
- Better characterization of factors that determine host range and virulence in cell culture and experimental animal infections.
- Poxvirus species leaping is still in its infancy. Natural reservoirs and the risk of establishment of new reservoirs in novel animal hosts during widespread outbreaks.
- Comprehensive understanding of cellular mechanisms promoting or inhibiting poxvirus adaptation for zoonotic infection and “species leaping.”
Other
- Identification of unknown poxviruses.
- Which poxviruses may pose the biggest risk to humans.
- Variants of oncolytic poxviruses that can tumor-target after intravenous delivery.
SOURCES: Personal communication, G. McFadden, Arizona State University retired, January 18, 2024; personal communication, Y. Xiang, UT Health San Antonio, January 21, 2024; personal communication, S. Rothenburg, University of California, Davis, January 24, 2024; personal communication, B. Moss, National Institute of Allergy and Infectious Diseases, January 18, 2024; personal communication, J. Liu, University of Arkansas for Medical Sciences, January 26, 2024.
could create novel bioterror agents that render an established MCM ineffective. Beneficial uses, if strategically developed and promulgated, could significantly mitigate infectious diseases as threats to personal, public, political, and environmental health.
Some argue that the continued existence of live VARV poses obvious inherent risks, including accidental release, laboratory-acquired infections, and the deliberate misuse of the virus as a bioterrorism weapon. Efforts to mitigate these risks involve stringent biosafety and biosecurity measures, strict multilateral global regulation and oversight of all research with live virus, and collaboration and transparent communication with the public and the international community. The exact extent of the risk entailed by keeping live virus and the effectiveness of various efforts to mitigate these risks are challenging to assess, while the potential benefits of research using live virus at the current time are easier to quantify.
Potential Risks of Technological Advancements on MCM Readiness
Synthetic Biology and Gene Editing
Advancements in the fields of synthetic biology and reverse genomics have shown that infectious viruses (e.g., horsepox virus and SARS-CoV-2) can be constructed in advanced laboratory settings based on published genomes (Jaschke et al., 2019; Levrier et al., 2023; Noyce et al., 2018; Thi Nhu Thao et al., 2020). This suggests that infectious variola virus, or a virus resembling variola virus, could similarly be re-constructed (Impelluso and Lentzos, 2017). Modern risk-reduction strategies must therefore account for the additional risk posed by synthetic biology and gene editing, such as by ensuring that biosecurity regulations for research with gene sequences of VARV keep pace with the increasingly available and lower-cost technology that can be used to produce such sequences (WHO, 2015).
Member companies of the International Gene Synthesis Consortium have voluntarily committed to screening DNA synthesis orders for sequences aligned with dangerous pathogens, such as those on the U.S. Select Agent and Toxin List1 and the Australia Group Common Control List (Hoffmann et al., 2023). The United States has historically not required such screening for orders, but could, and the Department of Health and Human Services (HHS) issued guidance in 2010 recommending baseline standards for the gene synthesis industry to ensure compliance with Select Agent Regulations, Export Administration Regulations, and, in general,
___________________
1 Federal Select Agent List, https://www.selectagents.gov/sat/list.htm (accessed February 5, 2024).
best practices for addressing biosecurity concerns (HHS, 2010). In February 2024, the International Biosecurity and Biosafety Initiative for Science was launched and intends to collaborate with governments, nongovernmental organizations, industry, and academia to develop shared practices and tools for international bioscience and biotechnology (e.g., cost-effective screening software for DNA synthesis providers) (NTI, 2024). The Biden administration has issued an executive order tasking the Office of Science and Technology Policy to issue a framework to encourage providers to implement comprehensive screening mechanisms—but it also requires all federal agencies that fund life science research to establish conditions of funding related to synthetic nucleic acid procurement through providers that adhere to the framework (White House, 2023). Stronger legal regulation of gene synthesis could be possible and might appear desirable if access to gene synthesis tools becomes widely availability outside of government-funded labs. The real-world effectiveness of stronger DNA synthesis regulations on preventing mal-intentioned actors from acquiring smallpox or other pathogens by synthesis is unknown and has been called into question (Carlson, 2015).
Regardless, smallpox is unique among pathogens when it comes to the governance of gene synthesis in the United States because possession or work with the virus itself is already subject to stringent regulations. In 2004—when concern over smallpox and other agents that could be used as bioweapons was heightened—Congress passed a terrorism prevention law, signed by President Bush. Title 18, Section 175c of the U.S. criminal code has stated ever since that it is “unlawful for any person to knowingly produce, engineer, synthesize, acquire, transfer directly or indirectly, receive, possess, import, export, or use, or possess and threaten to use, variola virus” (with an exception for work conducted “by, or under the authority of” the HHS secretary).2 The law goes on to define variola virus as the virus itself or any derivative that contains more than 85 percent of the gene sequence of the variola major or variola minor virus. Variola is the only pathogen that has such an entry in the code. Thus, law enforcement in the United States is already empowered to deter, investigate, and intervene on research or work involving smallpox—and there have been no reported violations of this law in the United States.
While construction of orthopoxvirus from scratch is now possible, the committee estimated that the number of labs capable of carrying out such work is limited to perhaps less than 100 globally. The committee expects this number to increase over the next two decades as DNA synthesis and genome construction techniques improve dramatically. Moreover, the modification of an existing orthopoxvirus to increase virulence has long been possible (Tucker, 2002). Thus, there is an urgent window of opportunity
___________________
2 18 U.S. Code § 175c—Variola virus (2004).
open now to use both policy and regulatory levers and emerging biotechnologies in developing and deploying next-generation solutions (below) for securing against any potential threats due to increasingly widespread access to synthetic biology capabilities.
Artificial Intelligence
While artificial intelligence (AI) and large language models (LLMs) hold the promise of significant human benefit, these technologies might also pose potential risks to biosecurity and could increase the likelihood of unsanctioned research to re-create smallpox or a virus resembling smallpox (Kuiken, 2023; White House, 2023). While efforts are underway to ensure that LLMs cannot be used to create explicit instructions for planning and executing a biological attack, LLMs could provide assistance in planning the technical details of an attack or by identifying the knowledge to recreate a virus in a laboratory setting (i.e., “AI assistants”) (Mouton et al., 2023; Sandbrink, 2023). Another class of AI tools dubbed “biological design tools” also pose potential risk as these tools are trained on biological data and have the capability to create proteins, gene clusters, orgenomes, and it is likely they will have the capability to create more complex biological structures with time (Sandbrink, 2023).
It is not yet clear exactly how such tools may be used or the exact nature of the risks and opportunities resulting. For example, a “long-context language model for the generation of bacteriophage genomes” recently reported development of software that automatically generates bacteriophage-like genomes starting from natural genome sequences (Shao, 2024). Whether any of the so-computed “genomes” encode viable phage is unclear. The committee conducted an ad hoc independent bioinformatic examination of the resulting sequences, which appear to encode phage-like genomes (e.g., open reading frame patterns), but at least some of the resulting open reading frames have no homology to any known proteins. As a second example, Nguyen et al. (2024) reported a 7 billion parameter model with a context length of 131 kilobases capable of generating coding rich sequences over 500 kilobases. De novo generation of Cas-like, transposon-like, and genome-like sequences were reported although, as with Shao, no experimental tests of function were reported. The tool, Evo, also supported mutational and gene essentiality analysis. Taken together, foreseeable advances could increase the likelihood that an AI-based tool could help researchers create viable phage and perhaps other viruses, including pox viruses. Already these tools could also aid researchers in analyzing and understanding pox viruses, natural or otherwise.
However, the impacts of AI-based tools on pox virus research and planning may be limited by the number of available poxvirus genome
sequences. There are orders of magnitude more phage and bacteria genome sequences available for training AI models, suggesting potential policy opportunities regarding the sequencing and publishing of any additional poxvirus genome sequences. The convergence of AI, synthetic biology, and reverse genomics might eventually lead to a future where previously unimaginable orthopox viruses could be prototyped in an advanced laboratory setting. These same tools, if well developed and organized in advance, could make understanding and responding to any such virus much easier. Such possibilities seem likely to become more material throughout the 2020s, emphasizing that now is the time to develop robust, resilient, and agile approaches for protecting against malicious or inadvertent future smallpox outbreaks.
Genome Sequencing
Advances in genomic sequencing and computational molecular biology have afforded an improved understanding of the genetic relatedness and evolutionary history of orthopox viruses, though gaps remain. The complete genome sequence of a virulent smallpox strain was first published in 1994 (Massung et al., 1994). Since then, gene sequencing technology has become portable, more accessible, and connected to computing and internet systems facilitating access to this information. Entire genomes can be sequenced in hours instead of weeks. Next-generation sequencing, which is a high-throughput process that allows for the sequencing of genes or genomes in a short timeframe, has enormous potential for its applications for research and diagnostics (Qin, 2019). As of early 2024 the Centers for Disease Control and Prevention (CDC) in the United States and the State Research Center of Virology and Biotechnology (VECTOR) in the Russian Federation have reported complete genome sequencing for, respectively, 40 and 50 smallpox isolates from their collections (WHO, 2023c). At the January 2024 meeting of the WHO executive board the representative of the Russian Federation called attention to potential concerns about making such sequences publicly available (WHO Executive Board, 2024). Thus, like many other technologies, genomic sequencing tools carry dual use potential (i.e., the benefits of learning about genomic variability across smallpox isolates come with the potential harm that sequencing these isolates is a first step toward creating them de novo using the tools below) (NASEM, 2017).
Potential Benefits of Technological Advancements on MCM Readiness
Many innovations in next-generation approaches to infectious disease diagnostics, vaccinations, and treatments, which might have utility in responding to a smallpox outbreak, have been developed around other
pathogens. It is important for such innovations to be evaluated and adopted, as useful, for mitigating risks associated with smallpox. As discussed in Chapter 2, isothermal amplification technologies were improved on during the COVID-19 pandemic and 2022 mpox outbreak as a more cost efficient, though less specific, alternative to quantitative polymerase chain reaction (qPCR) (Kaminski et al., 2021). Clustered regularly interspaced short palindromic repeats (CRISPR)-based diagnostics have also been developed to potentially address the need for diagnostic technologies that are cost efficient and maintain diagnostic accuracy (Kaminski et al., 2021). The potential for decentralized biomanufacturing vaccines can be further advanced via the use of lyophilized cell-free extracts (Warfel et al., 2023). More recent developments also suggest that naturally competent microbes can be bioengineered to serve as living sentinels to record the presence of tumors and viral species with health implications in both the environment and people (Cooper et al., 2023; Nou and Voigt, 2024).
Whole-cell synthetic biology has resulted in breakthrough approaches to metabolic engineering, including yeast-based production of complex natural medicinal products (e.g., scopolamine, hyoscyamine) (Srinivasan and Smolke, 2020). As a result, such active pharmaceutical ingredients and biosynthetic key starting materials can be quickly manufactured at scale, without depending on complex synthetic chemistry or agriculture-based supply chains (Antheia, 2024). This suggests that it may be strategically possible to develop future smallpox treatments that can be biosynthesized.
While many questions regarding synthetic mRNA vaccines should be explored (e.g., why immunity is short lived for COVID), next-generation approaches to vaccination continue to be developed. For example, commensal skin microbes have recently been modified to present antigens to the immune system, resulting in initial protection against melanoma in mice (Chen et al., 2023). If such approaches could be developed for smallpox, then vaccine manufacturing and readiness would become much easier to manage.
Taken together, emerging biotechnologies promise rapid, reliable, and local production of diagnostics, treatments, and vaccines. If this comes to pass, then future readiness could be less oriented around maintaining massive physical stockpiles of finished products and more focused on developing and proving the capacities to respond and deploy on demand, when and where needed (Hepburn, 2023). Realizing this future would require significant strategic leadership, coordination, and investment.
The COVID-19 pandemic and the mpox outbreak spurred significant research and development efforts for vaccines, biologics, therapeutics, and diagnostic tools. Lessons learned from these experiences can inform the evaluation of smallpox MCMs and illustrate the potential value of investing in innovative technologies.
A non-exhaustive list of additional tools that could help develop a more forward-looking approach to smallpox readiness includes:
- Artificial intelligence: for instance, protein structure prediction (alpha-fold) and bioinformatics. These AI technologies have enabled such advances as protein structure modeling used to understand proteins’ function and evolutional histories and to identify potential drug targets (Mutz et al., 2023).
- Genome sequencing: rapid growth in next-generation sequencing has made possible the rapid identification of pathogens, the monitoring of drug-resistance mutations and dominant strains/variants, and the suggestion of evolutionary mechanisms and directions, and has facilitated the epidemiologic tracking of transmission (GAO, 2021; Grad and Lipsitch, 2014; Jauneikaite et al., 2023).
- Proteomics: the study of the entire complement of proteins expressed by a virus has provided more in-depth understanding of viral structure and biology and may provide novel targets or strategies for MCMs (Zubair et al., 2022).
- Lipidomic profiling: similar to proteomics, lipidomics has elucidated the lipid profiles required for viral replication, which may suggest novel antiviral targets (Martín-Acebes et al., 2019; Yuan et al., 2019).
- Super-resolution microscopy and imaging: tools like cryogenic electron microscopy, cryo-electron tomography, and super-resolution imaging have the potential to identify antiviral targets with novel mechanisms of action.
- CRISPR: CRISPR-based antivirals could offer broad-spectrum therapeutics for all orthopoxvirus species (Mayes, 2023). CRISPR technology has also been applied to mpox diagnostics (Jiang et al., 2023).
Conclusions on Benefits and Risks of Scientific and Technological Advances
(3-4) The potential exists to synthesize the complete or partial variola virus genome and to manufacture infectious viral particles based on published genomes. Targeted modifications to the genome are also possible, which could alter functional components of the virus that could affect transmissibility or virulence. This capacity means that even the guaranteed complete eradication of all existing smallpox collections today would not guarantee against its re-emergence as a threat. It also introduces greater challenges in readiness planning by introducing the possibility of atypical epidemiological or clinical presentations of the disease.
(3-5) Advances in emerging biotechnologies could also allow for the rapid development and deployment of MCMs. A global, real-time, distributed, manufacturing network could enable safe and equitable production of smallpox diagnostics, vaccines, and therapeutics when and where needed to rapidly bring an outbreak anywhere in the world under control. A strategic research and development program promoting the development of general capability in this regard has the potential to unlock such a future.
OPERATIONAL CONSIDERATIONS FOR MCM READINESS AND RESPONSE
The U.S. MCM enterprise is a complex system that includes public and private entities that develop, manufacture, store, distribute, and administer MCMs. COVID-19 and mpox underscored the importance of an effectively coordinated MCM enterprise and highlighted significant gaps in federal readiness (NASEM, 2021). And while the smallpox portfolio is relatively mature compared to other products in the U.S. Strategic National Stockpile (SNS), these products have never been deployed in response to an actual smallpox outbreak so it is unknown how well the system will perform, to what extent smallpox MCMs will reach intended end users, and how frontline responders and laboratories will manage the influx of smallpox cases.
Manufacturing Capacity
While modern manufacturing of smallpox MCMs has advanced significantly since the “animal vaccine” farms of the late 19th century (Esparza et al., 2020), the SNS still relies on just a few manufacturers, so the failure of just one entity to deliver needed MCMs could undermine readiness and response. If the SNS administrators were to undertake an assessment of the proportion of the SNS’s smallpox MCM holdings produced by each company and how the loss of manufacturing capacity from any given company could affect readiness for full-scale population needs, it could inform decisions about whether investment from the Biomedical Advanced Research and Development Authority and SNS should be further diversified across more companies and other strategies to the ensure stability of the manufacturing base. One concerning situation could be the potential for a country to prohibit any export of MCM required to respond to a smallpox outbreak. To mitigate this scenario, the Administration for Strategic Preparedness and Response (ASPR) could consider options that would allow onshore manufacturing of products that normally originate outside the United States in such conditions. For example, a manufacturer could be required under contract to establish a relationship with a U.S.-based, large molecule contract development and manufacturing organization (CDMO) to produce additional product on their behalf in a global crisis.
For all smallpox MCMs, questions remain on whether there is sufficient capacity to scale MCM production in the event of a large-scale smallpox event, considering decades of supply chain disruption and shortages as well as the time-intensive regulatory processes that ensure safety and efficacy of medical products (NASEM, 2022a,b). For example, lengthy lead times to obtain raw materials are a critical issue for scaling manufacturing capacity. Emergent BioSolutions reported that if the company did not continually maintain raw materials, component parts, and existing staff, it could take upward of 2 years for additional ACAM2000 vaccines to become available following a procurement request (Sinclair, 2023). Further, while the actual manufacturing of vaccine may only take days, release testing can take up to 9 months to satisfy U.S. Food and Drug Administration (FDA) requirements (Chaplin, 2023).
The COVID-19 pandemic offers salient lessons for countermeasure availability. Once the pandemic began, the mechanism the U.S. government used was Operation Warp Speed (OWS). Enabled by a June 2020 memorandum of understanding between HHS and the Department of Defense (DoD), OWS was a joint effort to accelerate the development, production, and distribution of COVID-19 vaccines, which involved hundreds of staff from across the two departments (GAO, 2022). In January 2022, the Government Accountability Office (GAO) identified lessons learned from OWS. Among the positive decisions made by OWS, GAO cited investment diversification across multiple companies using different platform technologies and parallel investment in large-scale manufacturing while clinical trials were still ongoing (GAO, 2022).
Some options to ensure commercial manufacturing capability might include support for warm-base and just-in-time manufacturing (NASEM, 2016). Steps such as these can help foster vaccine availability at points of need during the initial period of identification of a smallpox outbreak. The commercialization of cross-protective orthopoxvirus MCMs would also be an important step: It would be attractive to industry, would inherently provide a warm base from which to support an emergency manufacturing response, and would ground a system of establishment and replenishment of vendor-managed inventory of cached vaccine (or raw vaccine material). The expected 2024 commercialization of MVA-BN for the purpose of controlling mpox in the United States, for instance, may enable greater availability of the vaccine for a smallpox emergency. Advances in the use of poxviruses as vaccine vectors, for gene delivery, and as oncolytic virotherapy might also bring benefits for enhancing smallpox MCMs, and these might also attract commercial resources and therefore enhance the smallpox response capability.
In 2012, HHS established the Centers for Innovation in Advanced Development and Manufacturing (CIADM), composed of three physical
manufacturing sites, to improve domestic infrastructure and expertise to produce MCMs in response to public health emergencies. Among other challenges, the program lacked sustained funding and regular manufacturing work to prepare for a real-world response and, during the COVID-19 pandemic, faced challenges with reliably producing products at scale (GAO, 2023). HHS has ended the CIADM program and is transitioning to a new national Biopharmaceutical Manufacturing Preparedness Consortium (BioMaP), a consortium-based approach of industry partners working together with government through flexible contracting authorities to expand the industrial and manufacturing base. It is not entirely clear how HHS will avoid some of the same challenges that faced CIADM, and the new model will require appropriate and sustained funding, rapid federal agency contracting capacity, and relevant expertise. In 2020, DoD granted the BioIndustrial Manufacturing and Design Ecosystem (BioMADE) an award for a new Manufacturing Innovation Institute to collaborate with public and private entities and advance sustainable manufacturing capabilities for the U.S. bioindustrial base (DoD, 2020).
Access and Uptake
The successes of OWS in developing safe and effective vaccines for COVID-19 in record time were tempered by major issues with accessibility and uptake domestically and globally. The billions of U.S. dollars invested in research and development for COVID-19 vaccines helped turn the tide against serious illness and hospitalization, while also revealing a level of vaccine hesitancy and differential uptake across the United States that had not been planned for (CDC, 2023; Kates et al., 2022; Robinson et al., 2022). In addition to hesitancy, uptake was also influenced by access. A 2022 Kaiser Family Foundation (KFF) analysis of Paxlovid and another oral therapy being used under an Emergency Use Authorization, Lagevrio (molnupiravir), found disparities in access to at-home orally administered treatments in the United States, with impoverished counties and individuals who were majority Black, Hispanic, or American Indian or Alaska Native facing reduced access—the same populations that disproportionately suffered poor COVID-19 outcomes (Hill et al., 2022; Leggat-Barr et al., 2021; Magesh et al., 2021) The potential role of effective therapeutics was a salient consideration in a pandemic characterized by a concerning level of vaccine hesitancy. Understanding the dynamic interplay between vaccine and treatment access and acceptance will be important for modeling uptake scenarios for smallpox MCMs.
While the nature and amounts of MCMs procured and stockpiled influence national infectious disease preparedness, comprehensive readiness necessitates a whole-of-society approach. The success of an MCM intervention rests on the successful delivery of the product and on sufficient uptake among
target or prioritized populations. COVID-19 revealed in stark terms that social, ethical, political, and market factors are all part of the fabric of readiness and resilience. It is especially concerning that several U.S. states have taken actions to limit vaccination requirements, which weakens the capacity of the public health system or the federal government to respond to future viral threats (National Academy for State Health Policy, 2024).
An operational or implementation research agenda could be invaluable in developing an improved understanding and better planning for smallpox MCM deployment. Components of such a research agenda might include developing strategies for rapidly collecting real-world evidence, improving turnaround times for testing, and equity-related parameters. This information could be critical to appropriate stockpile planning.
Vaccine Hesitancy and Risk Communications
While it is outside the scope of the committee’s charge to fully deliberate on the sociopolitical and economic factors that might influence stockpile decisions, the committee would like to highlight a few key dynamics that federal and state planners should consider in their decisions about smallpox readiness planning as they relate to downstream operational challenges.
Vaccine hesitancy is not a new phenomenon, but the national experience with COVID-19 and mpox demonstrated what vaccine hesitancy can mean for outbreak or pandemic response and how politics, policies, and misinformation can influence vaccine uptake. Planners must factor the potential role of vaccine hesitancy and other forms of mistrust of science, scientists, government, and industry into the predicted success of any smallpox strategy and seriously consider whether people will accept the risks associated with a replication-competent vaccine (see Chapter 1). Multiple studies have attempted to ascertain end-user acceptance of replicating smallpox vaccine. One study assessed health care provider willingness in 2002 to receive pre-event smallpox vaccination; 73 percent were willing, and the most common reason cited for those who did not want the vaccine was concern over adverse events (Everett et al., 2003). A second similar study yielded comparable results (Yih et al., 2003). But hesitance to receive the vaccine in the past (and today) may be related to the fact that smallpox is not in active circulation so it is reasonable to consider that uptake willingness might differ in a post-outbreak scenario. A 2004 analysis of differential willingness within the general public to undergo smallpox vaccination found that 84 percent of respondents reported being willing to get vaccinated in a hypothetical post-exposure ring vaccination scenario, and that number held regardless of racial identification; in a pre-exposure scenario, the number was substantially higher in White participants (77 percent) than African American (54 percent) (Micco et al., 2004).
What all of this indicates is that communicating the risk and benefits of smallpox vaccination versus infection, which carries far higher rates of disfiguration or death than does vaccination, will be critically important. But in the cases of COVID-19 and mpox, effective risk communication has been a struggle, especially in countering vaccine hesitancy driven by misinformation and disinformation about vaccines and the politicization of vaccination. These same challenges could occur in a smallpox outbreak and may be exasperated by other factors such as the origin of the smallpox event (e.g. laboratory accident or bioterror event) and who might be implicated in such an event (e.g., non-state actors or the laboratories that maintain the remaining live variola viruses in the United States and Russia). And while social and traditional media platforms will be able to quickly share images of smallpox patients from the 1960s and 1970s—showing the severity of the disease and hopefully encouraging intended populations to take protective measures—these same platforms could be used by actors to also propagate misinformation, disinformation, and altered imagery. Lessons learned about addressing misinformation, such as by enlisting locally trusted messengers, following public health risk communication principles (e.g., CDC’s Crisis and Emergency Risk Communication program) and misinformation management strategies (CDC, 2018; Nagar et al., 2024), and learning from previous smallpox vaccine campaigns (IOM, 2005), could be critical to an effective risk communication response. Prepositioned statements and guidelines for smallpox prevention and treatment, including discussion about which interventions will likely be ineffective, could also be considered.
CDC has sample smallpox alert messages available on its website for state and local health departments to reference, but today these messages say only that smallpox is a “serious, life-threatening disease,” with no further information about smallpox morbidity and mortality (CDC, 2017b). Variables that might affect uptake will include individual- and population-level willingness to receive vaccine; the level of risk messaged to the public; whether the vaccine is being administered pre- or post-exposure; the level of tolerance for vaccine side effects (especially because there are known severe adverse events of replicating smallpox vaccine); the dosing regimen (one versus two doses); and acceptance of old versus new vaccine technologies. Historical lessons from smallpox, polio, and measles vaccination campaigns, for example, show that vaccine hesitancy can be diminished when trusted community leaders guide vaccination efforts, individuals feel invested in vaccine development, and vaccination efforts are integrated with broader public health efforts (Eddy et al., 2023). Recent experiences with mpox highlight issues around vaccine hesitancy, access and availability, and disease stigma (Agroia et al., 2023; Mektebi et al., 2024; Moawad et al., 2023). Community anxiety and mental health implications of a disease
outbreak and the public health response could also affect vaccine uptake and other health-related behaviors (Serafini et al., 2020) Overall, the public health community must gain the public trust by communicating clearly about the benefits, risks, and uncertainties in the deployment of vaccines and other MCMs.
Access to and uptake of COVID-19 countermeasures and concomitant health outcomes were often drawn along sociodemographic lines. Combinations of such factors as mistrust, geographic, financial, and cultural barriers to access, and language barriers led to differential access to and uptake of COVID MCMs by different ethnic, racial, geographic, political and other groups (Abba-Aji et al., 2022; Hill et al., 2022). These differences often led to starkly different health outcomes, stratified along racial, ethnic, and socioeconomic lines (Magesh et al., 2021). The wealth of studies on these dynamics can inform smallpox planning efforts and improve understanding about the:
- root causes of MCM hesitancy and how to evaluate and implement strategies to address hesitancy and how vaccine hesitancy relates to therapeutic hesitancy,
- role of transparency and science literacy in building trust in advance,
- role of avoiding or mitigating financial conflicts of interest in research on MCM development and production (IOM, 2009a), and
- planning for and anticipating public uptake based on the lessons learned from COVID-19 and mpox vaccine hesitancy and misinformation impacts (WHO, 2022).
Frontline Readiness and Biosafety
The COVID-19 and mpox experiences showed the importance of ensuring those on the front line—health care providers, public health practitioners and laboratorians, and first responders—have the capabilities and capacities to effectively and equitably diagnose, prevent, and treat in the event of an infectious disease outbreak. Jurisdictions must be prepared to receive vaccines, maintaining cold chain protocols, and preparing to repackage and further distribute (Adams, 2023).
According to IOM (2005), preparing key responders for smallpox does not necessarily involve vaccinating responders, but would ideally involve receive timely training and education to meet the demands of the situation. As mentioned in Chapter 2, smallpox is a disease that few clinicians in practice today have ever seen, and pox-like, lesion-causing diseases can be difficult to distinguish from each other (e.g., chickenpox and mpox have been mistaken for each other on initial evaluation, and one might expect similar initial confusion in a smallpox outbreak) (Leung, 2019).
Clinician readiness today requires sustaining a suspicion for the possibility of smallpox in patients with fever and a rash who test negative for other common pathogens. Once a single case is detected however, very rapid and intense engagement with the clinical community would be critical, including around diagnostic testing criteria and to provide up-to-date knowledge of the correct techniques for specimen collection and submission. The scope of clinician involvement would be related to the breadth of the outbreak. A small outbreak from an unintentional event, such as a lab accident, would be more contained and require a limited engagement of the local health care workforce and activation of appropriate regional or national quarantine and isolation facilities. An intentional release in a major population center, by contrast, would be a major health security event of immediate international concern, requiring mobilization across the health care system and the need to increase national and international readiness for possible further attacks. A large-scale national response would likely arise even if the initial number of cases were to be relatively limited, as illustrated by the U.S. response to the 2001 Anthrax letter attacks and the 2014–2016 Ebola cases. Furthermore, ongoing and intense engagement with clinicians, health systems, and first responders would be critical for optimizing public uptake of medical countermeasures, as noted above.
Since there are currently no point-of-care tests for smallpox, clinical recognition of smallpox among health care professionals will be extremely important during an outbreak. CDC has clinical guidance and evaluation flow charts available on its website (CDC, 2016, 2017a). Notably, CDC advises that health care providers use only personnel who have been vaccinated against smallpox to administer smallpox vaccines and to vaccinate exposed personnel within 72 (though preferably within 24) hours of their first exposure to a confirmed smallpox patient (CDC, 2017a). ASPR has indicated that shipping of smallpox MCMs from the SNS will begin within 8 hours after receiving the direction to deploy (Adams, 2023).
Clinical guidance for smallpox MCMs would need to be communicated to frontline providers. For example, the administration of replicating smallpox vaccine requires a multiple puncture technique that is different from that of other vaccines, and those who might need to deliver the vaccines may not be trained in this technique. Absent the application of this technique, the vaccine-neutralizing antibody titer mounted is minimal. The method, although easy to learn, causes pause for some clinicians, as it necessitates the appearance of blood spots where the bifurcated needle is used. A national program to train vaccinators in this technique at the local level would need to be rapidly implemented in the event of a smallpox outbreak, taking lessons from the national smallpox vaccination campaign that took place 2002–2003 (IOM, 2005). The most recent clinical guidance for smallpox
vaccine use was published nearly a decade ago in 2015 and only provides guidance for a post-event vaccination program (Petersen et al., 2015). This guidance should be updated to reflect new data on smallpox vaccines—especially data about use for prevention of mpox and its efficacy—and clinical guidelines for indications and use of smallpox therapeutics should be developed. For example, frontline providers would also need rapid, situation-based training on the use of replicating and non-replicating vaccines and other updated clinical use guidance.
Strict and comprehensive biosafety measures must also be in place to manage a smallpox emergence, but there are only a handful of locations in the United States certified and equipped to intake smallpox patients (i.e., the National Special Pathogen System), and these would likely be rapidly overwhelmed in a large outbreak. Rapid training on special pathogen handling at facilities in an outbreak location would therefore be necessary. Even then, it is unclear whether testing facilities and the health care system would have the capacity to manage smallpox samples and patients safely and securely. In this regard, investments in developing strategies and infrastructure to carry out rapid regional trainings for outbreak response would improve smallpox (and other pathogen) preparedness. The nation’s laboratory system should also be prepared to process smallpox samples. Opportunities to strengthen national laboratory systems based on experience from recent public health emergencies are described in Box 3-3. CDC reports that Laboratory Response Network (LRN) sites could be expanded to perform variola-specific testing if confirmatory testing is needed on a larger scale. As discussed in Chapter 2, diagnostics assays for variola are only available at select LRN labs due to biosafety considerations. This could result in low testing capacity at the beginning of a smallpox outbreak, not unlike the testing experiences at the start of COVID-19 and mpox in the United States. Smallpox is designated as a biosafety level 4 agent, so only a select number of laboratories could safely and securely process smallpox assays during the beginning of an outbreak. Furthermore, the LRN testing algorithm for smallpox is complex, and better suited for the detection of initial cases in the absence of smallpox than situations where disease is more established or widespread (King, 2023). WHO manages the biosafety inspections for the laboratories working with variola, which must comply with strict biosafety regulations (WHO, 2024b). In the event of a larger outbreak, an approach to expanding testing sites would have to take into consideration clinician recognition and familiarity with disease presentations, availability of potential technological advances including testing platforms or inactivation methods that allow tests to be performed at lower biosafety levels, increased biosafety and biosecurity training, and greater input from regulatory authorities regarding approval and certification processes (see below). Box 3-3 discusses potential solutions for smallpox testing issues.
BOX 3-3
Opportunities to Strengthen National Laboratory Systems for Smallpox
Biosafety
- Collaborating with commercial laboratories for specimen transport during public health emergencies.a
Test Development
- Providing redundancy in the initial test development process using advanced public health laboratories.a
Test Manufacturing
- Proactively developing government contracts with test manufacturers for supplies.a
Stockpiling
- Robustly stocking the Strategic National Stockpile with testing kits, test components, and other related supplies.b
Testing Capacity
- Updating current LRN assays by multiplexing and adapting to high throughput.*
- Establishing guidelines to ensure consistency and predictability among traditional and nontraditional health care testing settings.b
- Striving toward immediate access to validated methods, the ability to rapidly develop methods, and a trained testing workforce.b
- Securing federal testing capacity by designating laboratories, medical centers and test manufacturers to respond during outbreaks.c
- Establishing emergency funding mechanisms before outbreaks can greatly impact mobilization efforts and testing readiness.c
Data Management
- Developing a minimum dataset for the test request process and case definition.a
- Standardizing laboratory information management systems to ensure data can be shared within the national laboratory system.b
Regulatory
- Collaborating with FDA to develop a portfolio of pre-vetted test protocols to speed regulatory test approval in an emerging biological crisis.a
- Aspiring toward nationwide mandatory reporting requirements to coordinate mitigation efforts.b
- Need for federal testing guidelines with enforcement and guidance structures.b
- Creating and updating test protocols that are pre-reviewed by FDA could speed regulatory test approvals during public health emergencies.c
Overall
- Collaborating across public and private fields to address current deficits in laboratory systems and ensure that rapid scale-up of testing is possible during large-scale emergencies.b
- Coordinating and advancing testing preparation across public health laboratories, hospitals, academic medical centers, commercial laboratories, health care providers, and patients is necessary in order to optimize capacities and contributions.
NOTES: Source attribution indicated by the following:
c Brown Pandemic Center (2023).
SOURCES: Brown Pandemic Center (2023); King (2023); NASEM (2023).
Drawing on information gathered by the committee and lessons from the testing experiences for COVID-19 and mpox, Box 3-3 lists opportunities for improving smallpox laboratory testing.
Regulatory Readiness
As noted in Chapter 1, access to smallpox MCMs in both domestic and global stockpiles depends considerably on the regulatory status of existing and new MCMs as well as on risk–benefit calculations that must be carried out with incomplete information. As such, these regulatory decisions reflect important scientific, legal, and ethical considerations. The success of a regulatory system in approving new drugs or new indications depends on the regulators’ capacity, and recent reports have shown that FDA and its international partners struggle to keep pace with new products and applications (NASEM, 2020a,b). Furthermore, global regulatory and legal capacity must also be sufficient to approve safe and effective MCMs quickly and efficiently in the event of a smallpox outbreak.
Regulatory readiness must take into account two intertwined elements: timeliness and the ability to expand. Both COVID and mpox revealed that a timely early response can be enabled by the availability of diverse products, including vaccines, diagnostics, and therapeutics. A rapid and agile regulatory response to approving or otherwise authorizing promising MCMs can also support timelines. For the ability to expand—that is, to
scale up manufacturing and the capacity to deliver those finished products to end users—public, private, and academic partners are necessary. Part of government readiness for MCMs is recognizing the importance of inclusion of other partners in virtually every aspect of preparedness. Supply chain issues are inevitable, for example, and if there are limited vendors for a particular MCM and there are quality issues, then downstream effects will be significant.
Regulatory decisions about the development and potential stockpiling and use of agents that have not yet been rigorously tested for safety and efficacy must balance multiple considerations. For instance, novel smallpox MCMs cannot be tested in humans with smallpox because the disease has been eradicated, but the use of MCMs developed for smallpox in the mpox outbreaks provided valuable proxy data indicating that they might have pan-orthopoxvirus applications (Dalton et al., 2023). There will always be some uncertainty about the cross-utility of MCMs within a viral family, but other orthopoxvirus outbreaks are considerably more likely than a smallpox outbreak, and investments in therapeutics targeting other orthopoxviruses might make it even more likely that these MCMs will have multiple uses and more immediate health benefits (i.e., will certainly be useful for other orthopoxvirus and might also be useful for smallpox).
At the point when a novel MCM is being developed and deployed, there is often a need to balance an understandable desire for rapid access to a promising new drug with ensuring that robust evidence is collected to determine if the product is in fact sufficiently safe and effective. Allowing an ineffective or harmful MCM to be deployed could raise false hopes of mitigation or, worse, cause significant harm to patients and whole populations. Yet delaying or disallowing authorization or approval of promising drugs until they are fully vetted would withhold potential benefits, especially for patients at greatest risk. Adhering to rigorous research ethics is essential even in the midst of a health emergency. In responding to COVID-19, for example, there has often been tension between providing rapid access (framed around respect for autonomy, “right to try,” or “compassionate use”) and conducting rigorous research to ensure that initially promising COVID MCMs are, in fact, safe and effective (Dominus, 2020). Efforts to navigate the competing values of rapid access versus rigorous research during the COVID-19 pandemic led, for example, to the initiation of platform trials in which every enrolled patient received a promising therapeutic (no one received placebo) (Macleod and Norrie, 2021). It also led to reassessments of the relative value of ongoing real-world evidence collection and strategies to improve the quality of this form of evidence as means of achieving this difficult balance (Schad and Thronicke, 2022).
These experiences from COVID-19 should be considered in planning for rapid MCM development, testing, and deployment in the event of a smallpox or other orthopoxvirus outbreak. For instance, the immediate
initiation of a platform trial to compare the effectiveness of available proposed smallpox MCMs could lead to very rapid determinations of real-world effectiveness, which could be facilitated by having a research protocol in place and approved prior to the event.
Key MCM Regulations for Smallpox Readiness and Response
Under section 564 of the Federal Food, Drug, and Cosmetic Act3, FDA may authorize unapproved medical products or uses during a public health emergency as declared by the Secretary of HHS under Emergency Use Authorization (EUA). All the COVID-19 vaccines were initially distributed under an EUA while their sponsors sought full approval. MVA-BN received FDA approval in 2019 for the prevention of smallpox and monkeypox disease in adults at high risk of infection. In 2022, however, faced with the realities of an outbreak that differed from the assumptions of the approval, FDA issued an EUA that expanded the population to those under 18 and permitted an alternate route of injection for adults (FDA, 2022a). Thus, while the SNS holds FDA-approved vaccines and treatments, an outbreak might necessitate the issuance of an EUA or an investigational new drug (IND) permit to use these in a different way. Such regulatory mechanisms could also enable the use of other products such as the Aventis Pasteur smallpox vaccine (see Chapter 2), which lacks FDA approval and would only be released under an EUA or IND “for use in circumstances where ACAM2000 is depleted, not readily available, or in a case-by-case basis where ACAM2000 is contraindicated” (CDC, 2022).
The emergency use of an unapproved investigational drug or biologic may require an IND (FDA, 1998). Emergency use is defined as the use of an investigational drug or biological product with a human subject in a life-threatening situation in which no standard acceptable treatment is available and in which there is not sufficient time to obtain institutional review board approval.4 As of this writing, tecovirimat may be requested for mpox patients under an expanded access IND (CDC, 2024).
Expiration dating extension.
Expiration dates of stockpiled MCMs can present challenges, and FDA is engaged in four approaches to extend expiration dates: initiated by the manufacturer, the shelf-life extension program, emergency use authorities, and enforcement discretion (FDA, 2024a). In February 2023, FDA approved a shelf-life extension for tecovirimat, a drug approved for smallpox and contained in the SNS, from 24 months to 42 months (FDA, 2024a).
Animal Rule.
To prove the efficacy of a product during circumstances in which human challenge studies would not be ethical or feasible, such as for smallpox, FDA may grant approval based on well-controlled animal studies
___________________
3 21 U.S.C. § 360bbb-3.
4 21 CFR 56.102(d).
and demonstration of the product’s safety in humans (FDA, 2023a). For example, tecovirimat was approved under FDA’s Animal Rule after showing effectiveness and efficacy only in in vitro and animal model studies, coupled with safety data in humans (CDC, 2021).
Regulatory flexibility.
Two federal regulations apply to laboratory testing for clinical use. The Clinical Laboratory Improvement Amendments regulate all laboratory testing (except research) performed on humans in the United States and it is overseen by the Centers for Medicare & Medicaid Services (CMS) (CMS, 2024). The second is the Food, Drug, and Cosmetic Act (FD&C) that applies to tests marketed for clinical use and is under FDA’s jurisdiction. CMS and FDA may exercise enforcement discretion for diagnostics authorized under a public health emergency (e.g., enforcement discretion was applied during COVID-19, so tests could be run on asymptomatic individuals, which went beyond authorization limited to symptomatic individuals). Early in the COVID-19 pandemic, emergency provisions in the FD&C were invoked, giving FDA the ability to grant EUAs for diagnostic tests prior to completing the normal review process. This allowed many commercially developed tests to be available faster to the consumer. However, the same provisions simultaneously introduced additional requirements for laboratory developed tests (LDTs) requiring sites, such as hospitals and universities, to obtain an EUA for their home-grown tests (FDA, 2022b; Sluzala and Haislmaier, 2022). FDA has generally exercised enforcement discretion over LDTs, which waived pre-market review requirements needed on commercially marketed diagnostic tests. The FDA stance was further articulated during the mpox pandemic, when the agency reverted to enforcement discretion policies for certain mpox tests developed by laboratories, as long as the LDTs were performed in a high complexity Clinical Laboratory Improvement Amendments (CLIA)-certified lab, and were not at-home tests or did not include home specimen collection (FDA, 2023b). This allowed LDTs for mpox to be used at non-LRN sites (i.e., clinical or academic centers) increasing the number of patient testing sites and bringing testing closer to the patient (Caldera et al., 2023; Conger, 2022, FDA, 2023b). Recently, FDA, with support from CMS, has signaled that it will move away from its historic enforcement discretion stance and provide more oversight of LDTs (FDA, 2024b). Further clarification from FDA and CMS on how they will approach regulatory flexibility during future public health emergencies will be important factors in expanding access to testing and mitigating a smallpox outbreak of significant scale.
Relevant Ethical Questions for Smallpox MCMs
Basic science research on smallpox may fit the definition of dual use research of concern and therefore receives additional ethical scrutiny (NASEM, 2017). MCM research, by contrast, typically does not pose a direct dual use
threat, insofar as research on or stockpiling of a new therapeutic or vaccine would not entail learning about how to make the virus more virulent or infectious. But developing and stockpiling MCMs does pose a closely related ethical challenge. Namely, adversaries might consider U.S. plans to vaccinate or treat the populace as a threat in that it would reduce U.S. vulnerability to a smallpox bioweapon. U.S. scientists and public health planners might view as unlikely the potential for the United States to develop and use a smallpox bioweapon in breach of its legal and ethical obligations, but planners should not assume all actors would have such reservations and others might not trust the United States to hold to its commitments. For this reason, strong and verifiable international agreements about resource and knowledge sharing, international collaborations, and transparency all hold special importance in the arena of smallpox MCM development and stockpiling.
Conclusions on Factors that Influence Readiness and Response Posture
(3-6) The small number of manufacturers of smallpox MCMs is a readiness and response vulnerability—and it is clear there is insufficient capacity to scale MCM production in the event of a large-scale smallpox event especially one of international scope.
(3-7) Given the lack of commercially available orthopoxvirus diagnostics, vaccines, and therapeutics, planning for logistics and supply chain management considerations is critical. Efforts could give consideration to developing plans to increase the number of smallpox vaccine and therapeutics manufacturers as well as optimizing current manufacturing capacities should they be needed in the shorter term.
(3-8) Communicating the risk and benefits of smallpox vaccination versus infection will be critically important. But experience with COVID-19 and mpox demonstrated that effective risk communication has been a challenge, especially considering vaccine hesitancy and the politicization of vaccination, and misinformation and disinformation. These same challenges could occur in a smallpox outbreak.
(3-9) Implementation research investigating the operational and social aspects of deploying and uptake of smallpox MCMs is needed to assess operational parameters that could affect readiness and response.
(3-10) Those on the front line—health care providers, public health practitioners and laboratorians, and first responders—need to have the capabilities and capacities to effectively and equitably diagnosis, prevent, and treat in the event of a smallpox outbreak. Clinical and public health guidance should be updated to reflect new data and new MCMs and should take into consideration the range of response strategies beyond post-exposure programs (i.e., ring vaccination).
(3-11) Regulatory readiness and responsiveness, applicable to all types of MCMs, will be critical in the event of a smallpox outbreak. This is especially relevant considering the additional laboratory biosafety concerns for smallpox compared with other orthopoxviruses.
(3-12) New regulatory models that can quickly evaluate MCMs that use novel platforms and newer methodologies need to be developed and implemented. This could be achieved through the sharing of necessary product characteristics, detailed submission requirements, and setting accepted benchmarks and immune assays (in the case of vaccines) ahead of time, as well as planning for surge staffing to ensure timely review and real-time engagement for inquiries.
OVERARCHING CONCLUSIONS
Based on the evidence and findings on the ongoing utility of orthopoxvirus research more broadly for smallpox readiness and response, on the implications of scientific and technological advancements on available smallpox MCMs, and on operational considerations affecting SNS planning, the committee drew the following overarching conclusions:
In addition to smallpox readiness, research should continue to be used to enhance readiness and response for other orthopoxviruses, this includes supporting the validation, approval and licensure, and commercialization of existing and next-generation MCMs for use in the management of non-variola orthopoxviruses as an efficient way to expand readiness more broadly by enabling vendor-managed inventory approaches to stockpiling.
A comprehensive and ongoing risk–benefit analysis is needed for smallpox MCMs research using emerging technologies as well as ongoing careful oversight to mitigate the risks of this research and ensure the risk–benefit balance is maintained.
Readiness and response efforts involving MCMs are complex due to many factors. MCM development, stockpiling, and distribution planning must be flexible, adaptable, and robust against multiple potential smallpox event scenarios. Planning strategies should account for the complexities of each scenario and aim to support several health and well-being outcomes (e.g., health, justice, equity, and national/international demand).
REFERENCES
Abba-Aji, M., D. Stuckler, S. Galea, and M. McKee. 2022. Ethnic/racial minorities’ and migrants’ access to COVID-19 vaccines: A systematic review of barriers and facilitators. Journal of Migration and Health 5:100086.
Adams, S. A. 2023. Strategic National Stockpile smallpox medical countermeasures overview. Presentation at Meeting 3 of the Committee on Current State of Research, Development, and Stockpiling of Smallpox MCMs of the National Academies. December 14. https://www.nationalacademies.org/event/41411_12-2023_meeting-3-of-the-committee-on-the-current-state-of-research-development-and-stockpiling-of-smallpox-medical-countermeasures (accessed February 18, 2024).
Agroia, H., E. Smith, A. Vaidya, S. Rudman, and M. Roy. 2023. Monkeypox (mpox) vaccine hesitancy among mpox cases: A qualitative study. Health Promotion Practices December 15:15248399231215054.
Alaska Department of Health. 2024. Alaskapox virus. https://health.alaska.gov/dph/Epi/id/Pages/Alas-kapox.aspx (accessed January 22, 2024).
Antheia. 2024. Antheia completes successful product validation. PR Newswire, https://www.prnewswire.com/news-releases/antheia-completes-successful-product-validation-302026695.html (accessed February 5, 2024).
Antinori, S., G. Casalini, A. Giacomelli, and A. J. Rodriguez-Morales. 2023. Update on mpox: A brief narrative review. InfezMed 31(3):269–276.
Baylor, N. W., and J. L. Goodman. 2022. Vaccine preparedness for the next influenza pandemic: A regulatory perspective. Vaccines (Basel) 10(12):2136.
Berche, P. 2022. Life and death of smallpox. La Presse Médicale 51(3):104117.
Bolislis, W. R., M. L. de Lucia, F. Dolz, R. Mo, M. Nagaoka, H. Rodriguez, M. L. Woon, W. Yu, and T. C. Kühler. 2021. Regulatory agilities in the time of COVID-19: Overview, trends, and opportunities. Clinical Therapeutics 43(1):124–139.
Bray, M., and M. Buller. 2004. Looking back at smallpox. Clinical Infectious Diseases 38(6):882–889.
Breman, J. G., and D. A. Henderson. 2002. Diagnosis and management of smallpox. New England Journal of Medicine 346(17):1300–1308.
Broad, W. J., and J. Miller. 2002. Traces of terror: The bioterror threat; report provides new details of Soviet smallpox accident. The New York Times, June 15.
Brown Pandemic Center. 2023. Testing playbook for biological emergencies. Providence, RI: Brown University.
Burrell, C. J., C. R. Howard, and F. A. Murphy. 2017. Chapter 16: Poxviruses. In C. J. Burrell, C. R. Howard and F. A. Murphy (eds.), Fenner and White’s medical virology (5th edition). London: Academic Press. Pp. 229–236.
Caldera, J. R., H. K. Gray, O. B. Garner, and S. Yang. 2023. FDA trial regulation of laboratory developed tests (LDTs): An academic medical center’s experience with mpox in-house testing. Journal of Clinical Virology 169:105611.
Carlin, E. P., N. Giller, and R. Katz. 2017. Estimating the size of the U.S. population at risk of severe adverse events from replicating smallpox vaccine. Public Health Nursing 34(3):200–209.
Carlson, R. 2015. Synthesis. http://www.synthesis.cc/synthesis/2015/05/brewing_bad_biosecurity_policy (accessed March 2, 2024).
CDC (U.S. Centers for Disease Control and Prevention). 2003. Update: Multistate outbreak of monkeypox—Illinois, Indiana, Kansas, Missouri, Ohio, and Wisconsin, 2003. Morbidity and Mortality Weekly Report 52(27):642–646.
CDC. 2016. Evaluating patients for smallpox. https://www.cdc.gov/smallpox/clinicians/algorithm-protocol.html (accessed February 6, 2024).
CDC. 2017a. Protect and care for smallpox patients. https://www.cdc.gov/smallpox/bioterrorism-response-planning/healthcare-facility/protect-care-patients.html (accessed February 6, 2024).
CDC. 2017b. Sample alert messages for the community. https://www.cdc.gov/smallpox/bioterrorism-response-planning/public-health/sample-alert-messages-community.html (accessed February 12, 2024).
CDC. 2018. Crisis & Emergency Risk Communication (CERC). https://emergency.cdc.gov/cerc (accessed February 28, 2024).
CDC. 2021. Treatment. https://www.cdc.gov/smallpox/clinicians/treatment.html (accessed February 7, 2024).
CDC. 2022. Vaccines. https://www.cdc.gov/smallpox/clinicians/vaccines.html (accessed February 7, 2024).
CDC. 2023. COVID data tracker. https://covid.cdc.gov/covid-data-tracker/#datatracker-home (accessed February 28, 2024).
CDC. 2024. Tecovirimat (TPOXX) for treatment of mpox. https://www.cdc.gov/poxvirus/mpox/clinicians/obtaining-tecovirimat.html (accessed February 12, 2024).
Chaplin, P. 2023. Current state of research, development, and stockpiling of smallpox MCMs. Presentation at Meeting 3 of the Committee on Current State of Research, Development, and Stockpiling of Smallpox MCMs of the National Academies. December 14. https://www.nationalacademies.org/event/41411_12-2023_meeting-3-of-the-committee-on-the-current-state-of-research-development-and-stockpiling-of-smallpox-medical-countermeasures (accessed February 18, 2024).
Chen, Y. E., D. Bousbaine, A. Veinbachs, K. Atabakhsh, A. Dimas, V. K. Yu, A. Zhao, N. J. Enright, K. Nagashima, Y. Belkaid, and M. A. Fischbach. 2023. Engineered skin bacteria induce antitumor T cell responses against melanoma. Science 380(6641):203–210.
CMS (Centers for Medicare & Medicaid Services). 2024. Clinical Laboratory Improvement Amendments (CLIA). https://www.cms.gov/medicare/quality/clinical-laboratory-improvement-amendments (accessed February 15, 2024).
Conger, K. 2022. Mpox test launched at Stanford Medicine to help combat global outbreak. https://med.stanford.edu/news/all-news/2022/06/monkeypox-test.html (accessed February 29, 2024).
Cooper, R. M., J. A. Wright, J. Q. Ng, J. M. Goyne, N. Suzuki, Y. K. Lee, M. Ichinose, G. Radford, F. J. Ryan, S. Kumar, E. M. Thomas, L. Vrbanac, R. Knight, S. L. Woods, D. L. Worthley, and J. Hasty. 2023. Engineered bacteria detect tumor DNA. Science 381(6658):682–686.
Costa, T., M. F. Stidworthy, R. Ehmann, D. Denk, I. Ashpole, G. Drake, I. Maciuca, G. Zoeller, H. Meyer, and J. Chantrey. 2023. Cowpox in zoo and wild animals in the United Kingdom. Journal of Comparative Pathology 204:39–46.
Dalton, A. F., A. O. Diallo, A. N. Chard, D. L. Moulia, N. P. Deputy, A. Fothergill, I. Kracalik, C. W. Wegner, T. M. Markus, P. Pathela, W. L. Still, S. Hawkins, A. T. Mangla, N. Ravi, E. Licherdell, A. Britton, R. Lynfield, M. Sutton, A. P. Hansen, G. S. Betancourt, J. V. Rowlands, S. J. Chai, R. Fisher, P. Danza, M. Farley, J. Zipprich, G. Prahl, K. A. Wendel, L. Niccolai, J. L. Castilho, D. C. Payne, A. C. Cohn, and L. R. Feldstein. 2023. Estimated effectiveness of jynneos vaccine in preventing mpox: A multijurisdictional case-control study—United States, August 19, 2022–March 31, 2023. Morbidity and Mortality Weekly Report 72(20):553–558.
Damon, I. K., C. R. Damaso, and G. McFadden. 2014. Are we there yet? The smallpox research agenda using variola virus. PLOS Pathogens 10(5):e1004108.
Decker, M. D., P. M. Garman, H. Hughes, M. A. Yacovone, L. C. Collins, C. D. Fegley, G. Lin, G. DiPietro, and D. M. Gordon. 2021. Enhanced safety surveillance study of ACAM2000 smallpox vaccine among US military service members. Vaccine 39(39):5541–5547.
Dobrovolná, M., V. Brázda, E. F. Warner, and S. Bidula. 2023. Inverted repeats in the monkeypox virus genome are hot spots for mutation. Journal of Medical Virology 95(1):e28322.
DoD (Department of Defense). 2020. DOD approves $87 million for newest bioindustrial manufacturing innovation institute. Washington, DC: Department of Defense.
Dominus, S. 2020. The COVID drug wars that pitted doctor vs. doctor. The New York Times Magazine, August 5. https://www.nytimes.com/2020/08/05/magazine/covid-drug-wars-doctors.html (accessed February 7, 2024).
Durski, K. N., A. M. McCollum, Y. Nakazawa, B. W. Petersen, M. G. Reynolds, S. Briand, M. H. Djingarey, V. Olson, I. K. Damon, and A. Khalakdina. 2018. Emergence of monkeypox—West and Central Africa, 1970–2017. Morbidity and Mortality Weekly Report 67(10):306–310.
Eddy, J. J., H. A. Smith, and J. E. Abrams. 2023. Historical lessons on vaccine hesitancy: Smallpox, polio, and measles, and implications for COVID-19. Perspectives in Biology and Medicine 66(1):145–159.
Esparza, J., S. Lederman, A. Nitsche, and C. R. Damaso. 2020. Early smallpox vaccine manufacturing in the United States: Introduction of the “animal vaccine” in 1870, establishment of “vaccine farms”, and the beginnings of the vaccine industry. Vaccine 38(30):4773–4779.
Everett, W. W., S. E. Coffin, T. Zaoutis, S. D. Halpern, and B. L. Strom. 2003. Smallpox vaccination: A national survey of emergency health care providers. Academic Emergency Medicine 10(6):606–611.
FDA (U.S. Food and Drug Administration). 1998. Emergency use of an investigational drug or biologic: Guidance for institutional review boards and clinical investigators. https://www.fda.gov/regulatory-information/search-fda-guidance-documents/emergency-use-investigational-drug-or-biologic (accessed February 7, 2024).
FDA. 2022a. Fact sheet for healthcare providers administering vaccine: Emergency Use Authorization of JYNNEOS (smallpox and monkeypox vaccine, live, non-replicating) for prevention of monkeypox disease in individuals determined to be at high risk for monkeypox infection. https://www.fda.gov/media/160774/download (accessed February 15, 2024).
FDA. 2022b. Policy for monkeypox tests to address the public health emergency: Guidance for laboratories, commercial manufacturers and Food and Drug Administration staff. https://www.fda.gov/regulatory-information/search-fda-guidance-documents/policy-monkeypox-tests-address-public-health-emergency (accessed March 2, 2024).
FDA. 2023a. Animal rule information. https://www.fda.gov/emergency-preparedness-and-response/mcm-regulatory-science/animal-rule-information (accessed February 15, 2024).
FDA. 2023b. Monkeypox (mpox) and medical devices. https://www.fda.gov/medical-devices/emergency-situations-medical-devices/monkeypox-mpox-and-medical-devices#Laboratories (accessed February 6, 2024).
FDA. 2024a. Expiration dating extension. https://www.fda.gov/emergency-preparedness-and-response/mcm-legal-regulatory-and-policy-framework/expiration-dating-extension#mpoxsmallpox (accessed February 15, 2024).
FDA. 2024b. FDA and CMS: Americans deserve accurate and reliable diagnostic tests, wherever they are made. https://www.fda.gov/medical-devices/medical-devices-news-and-events/fda-and-cms-americans-deserve-accurate-and-reliable-diagnostic-tests-wherever-they-are-made (accessed March 2, 2024).
GAO (Government Accountability Office). 2021. Science & tech spotlight: Genomic sequencing of infectious pathogens (GAO-21-426SP). Washington, DC: Government Accountability Office.
GAO. 2022. HHS and DOD transitioned vaccine responsibilities to HHS, but need to address outstanding issues (GAO-22-104453). Washington, DC: Government Accountability Office.
GAO. 2023. Public health preparedness: HHS should plan for medical countermeasure development and manufacturing risks (GAO-23-105713). Washington, DC: Government Accountability Office.
Gong, Q., C. Wang, X. Chuai, and S. Chiu. 2022. Monkeypox virus: A re-emergent threat to humans. Virologica Sinica 37(4):477–482.
Grabenstein, J. D., and W. Winkenwerder, Jr. 2003. U.S. military smallpox vaccination program experience. JAMA 289(24):3278–3282.
Grad, Y. H., and M. Lipsitch. 2014. Epidemiologic data and pathogen genome sequences: A powerful synergy for public health. Genome Biology 15(11):538.
Gubser, C., S. Hué, P. Kellam, and G. L. Smith. 2004. Poxvirus genomes: A phylogenetic analysis. Journal of General Virology 85(1):105–117.
Hammarlund, E., M. W. Lewis, S. G. Hansen, L. I. Strelow, J. A. Nelson, G. J. Sexton, J. M. Hanifin, and M. K. Slifka. 2003. Duration of antiviral immunity after smallpox vaccination. Nature Medicine 9(9):1131–1137.
Hedberg, H., and A. Zink. 2024. Fatal Alaskapox infection in a Southcentral Alaska resident. State of Alaska Epidemiology Bulletin. https://epi.alaska.gov/bulletins/docs/b2024_02.pdf (accessed February 15, 2024).
Hepburn, M. 2023. Remarks on lessons learned and future considerations for smallpox preparedness and readiness. Presentation at Meeting 3 of the Committee on Current State of Research, Development, and Stockpiling of Smallpox MCMs of the National Academies. December 14. https://www.nationalacademies.org/event/41411_12-2023_meeting-3-of-the-committee-on-the-current-state-of-research-development-and-stockpiling-of-smallpox-medical-countermeasures (accessed February 18, 2024).
HHS (Department of Health and Human Services). 2010. Screening framework guidance for providers of synthetic double-stranded DNA. https://www.phe.gov/Preparedness/legal/guidance/syndna/Documents/syndna-guidance.pdf (accessed February 15, 2024).
Hill, L., S. Artiga, A. Rouw, and J. Kates. 2022. How equitable is access to COVID-19 treatments? KFF, June 23. https://www.kff.org/coronavirus-covid-19/issue-brief/how-equitable-is-access-to-covid-19-treatments (accessed February 15, 2024).
Hoffmann, S. A., J. Diggans, D. Densmore, J. Dai, T. Knight, E. Leproust, J. D. Boeke, N. Wheeler, and Y. Cai. 2023. Safety by design: Biosafety and biosecurity in the age of synthetic genomics. iScience 26(3):106165.
Impelluso, G., and F. Lentzos. 2017. The threat of synthetic smallpox: European perspectives. Health Security 15(6):582–586.
IOM (Institute of Medicine). 2005. The smallpox vaccination program: Public health in an age of terrorism. Washington, DC: The National Academies Press.
IOM. 2009a. Conflict of interest in medical research, education, and practice. Washington, DC: The National Academies Press.
IOM. 2009b. Live variola virus: Considerations for continuing research. Washington, DC: The National Academies Press.
Jacobs, B. L., J. O. Langland, K. V. Kibler, K. L. Denzler, S. D. White, S. A. Holechek, S. Wong, T. Huynh, and C. R. Baskin. 2009. Vaccinia virus vaccines: Past, present and future. Antiviral Research 84(1):1–13.
Jaschke, P. R., G. A. Dotson, K. S. Hung, D. Liu, and D. Endy. 2019. Definitive demonstration by synthesis of genome annotation completeness. Proceedings of the National Academy of Sciences 116(48):24206–24213.
Jauneikaite, E., K. S. Baker, J. G. Nunn, J. T. Midega, L. Y. Hsu, S. R. Singh, A. L. Halpin, K. L. Hopkins, J. R. Price, P. Srikantiah, B. Egyir, I. N. Okeke, K. E. Holt, S. J. Peacock, and N. A. Feasey. 2023. Genomics for antimicrobial resistance surveillance to support infection prevention and control in health-care facilities. The Lancet Microbe 4(12):e1040–e1046.
Jezek, Z., and F. Fenner. 1988. Human monkeypox. Vol. 17 in Monographs in virology. S.Karger AG.
Jiang, T., G. Li, R. Liu, J. Zhou, N. Gao, and J. Shen. 2023. Creating an ultra-sensitive detection platform for monkeypox virus DNA based on CRISPR technology. Journal of Medical Virology 95(7):e28905.
Kaminski, M. M., O. O. Abudayyeh, J. S. Gootenberg, F. Zhang, and J. J. Collins. 2021. CRISPR-based diagnostics. Nature Biomedical Engineering 5(7):643–656.
Kates, J., J. Tolbert, and A. Rouw. 2002. The red/blue divide in COVID-19 vaccination rates continues: An update. https://www.kff.org/policy-watch/the-red-blue-divide-in-covid-19-vaccination-rates-continues-an-update (accessed February 28, 2024).
Kennedy, R. B., and P. A. Gregory. 2023. Chapter 55 - smallpox and vaccinia. In Plotkin’s vaccines (eighth edition), edited by W. Orenstein, P. Offit, K. M. Edwards and S. Plotkin. Philadelphia: Elsevier. Pp. 1057–1086.
King, E. 2023. Lessons learned and future considerations for smallpox preparedness and readiness. Presentation at Meeting 3 of the Committee on Current State of Research, Development, and Stockpiling of Smallpox MCMs of the National Academies. December 14. https://www.nationalacademies.org/event/41411_12-2023_meeting-3-of-the-committee-on-the-current-state-of-research-development-and-stockpiling-of-smallpox-medical-countermeasures (accessed February 18, 2024).
Kuiken, T. 2023. Artificial intelligence in the biological sciences: Uses, safety, security, and oversight. Washington, DC: Congressional Research Service.
Leggat-Barr, K., N. Goldman, and F. Uchikoshi. 2021. COVID-19 risk factors and mortality among Native Americans. Demographic Research 45(39):1185–1218.
Leung, J., A. M. McCollum, K. Radford, C. Hughes, A. S. Lopez, S. A. J. Guagliardo, B. Nguete, T. Likafi, J. Kabamba, J. Malekani, R. Shongo Lushima, E. Pukuta, S. Karhemere, J. J. Muyembe Tamfum, M. G. Reynolds, E. Wemakoy Okitolonda, D. S. Schmid, and M. Marin. 2019. Varicella in Tshuapa Province, Democratic Republic of Congo, 2009–2014. Tropical Medicine & International Health 24(7):839–848.
Levrier, A., I. Karpathakis, B. Nash, S. D. Bowden, A. B. Lindner, and V. Noireaux. 2023. Pheiges, all-cell-free phage synthesis and selection from engineered genomes. bioRxiv 2023.2012.2007.570578.
Liu, J., S. Wennier, L. Zhang, and G. McFadden. 2011. M062 is a host range factor essential for myxoma virus pathogenesis and functions as an antagonist of host SAMD9 in human cells. Journal of Virology 85(7):3270–3282.
Macleod, J., and J. Norrie. 2021. PRINCIPLE: A community-based COVID-19 platform trial. The Lancet Respiratory Medicine 9(9):943–945.
Magesh, S., D. John, W. T. Li, Y. Li, A. Mattingly-app, S. Jain, E. Y. Chang, and W. M. Ongkeko. 2021. Disparities in COVID-19 outcomes by race, ethnicity, and socioeconomic status: A systematic review and meta-analysis. JAMA Network Open 4(11):e2134147.
Martín-Acebes, M. A., N. Jiménez de Oya, and J. C. Saiz. 2019. Lipid metabolism as a source of druggable targets for antiviral discovery against zika and other flaviviruses. Pharmaceuticals (Basel) 12(2):97.
Massung, R. F., L.-I. Liu, J. Qi, J. C. Knight, T. E. Yuran, A. R. Kerlavage, J. M. Parsons, J. C. Venter, and J. J. Esposito. 1994. Analysis of the complete genome of smallpox variola major virus strain Bangladesh–1975. Virology 201(2):215–240.
Mayes, C. 2023. CRISPR-based antivirals as broad-spectrum therapeutics. Presentation at Meeting 3 of the Committee on Current State of Research, Development, and Stockpiling of Smallpox MCMs of the National Academies. December 14. https://www.nationalacademies.org/event/41411_12-2023_meeting-3-of-the-committee-on-the-current-state-of-research-development-and-stockpiling-of-smallpox-medical-countermeasures (accessed February 18, 2024).
McInnes, C. J., I. K. Damon, G. L. Smith, G. McFadden, S. N. Isaacs, R. L. Roper, D. H. Evans, C. R. Damaso, O. Carulei, L. M. Wise, and E. J. Lefkowitz. 2023. ICTV virus taxonomy profile: Poxviridae 2023. Journal of General Virology 104(5). https://doi.org/10.1099/jgv.0.001849 (accessed March 2, 2024).
Mektebi, A., M. Elsaid, T. Yadav, F. Abdallh, M. Assker, A. Siddiq, R. Sayad, M. Saifi, and R. A. Farahat. 2024. Mpox vaccine acceptance among healthcare workers: A systematic review and meta-analysis. BMC Public Health 24(1):4.
Micco, E., A. D. Gurmankin, and K. Armstrong. 2004. Differential willingness to undergo smallpox vaccination among African-American and White individuals. Journal of General Internal Medicine 19(5 Pt 1):451–455.
Moss, B., and G. L. Smith. 2021. Chapter 16: Poxviridae: The viruses and their replication. In P. M. Howley and D. M. Knipe (eds.), Fields virology: DNA viruses, vol. 2 (7th edition). Philadelphia: Wolters Kluwer Health. Pp. 573–613.
Moawad, M. H.-E., A. M. Taha, D. Nguyen, M. Ali, Y. A. Mohammed, W. A. E.-T. Moawad, E. Hamouda, D. K. Bonilla-Aldana, and A. J. Rodriguez-Morales. 2023. Attitudes towards receiving monkeypox vaccination: A systematic review and meta-analysis. Vaccines 11(12):1840.
Mouton, C. A., C. Lucas, and E. Guest. 2023. The operational risks of AI in large-scale biological attacks: A red-team approach. Santa Monica, CA: RAND Corporation.
Mutz, P., W. Resch, G. Faure, T. G. Senkevich, E. V. Koonin, and B. Moss. 2023. Exaptation of inactivated host enzymes for structural roles in orthopoxviruses and novel folds of virus proteins revealed by protein structure modeling. mBio 14(2):e0040823.
Nagar, A., V. Grégoire, A. Sundelson, E. O’Donnell-Pazderka, A. M. Jamison, and T. K. Sell. 2024. Practical playbook for addressing health misinformation. Baltimore, MD: Johns Hopkins Center for Health Security.
NASEM (National Academies of Sciences, Engineering, and Medicine). 2016. Global health risk framework: Research and development of medical products: Workshop summary. Washington, DC: The National Academies Press.
NASEM. 2017. Dual use research of concern in the life sciences: Current issues and controversies. Washington, DC: The National Academies Press.
NASEM. 2020a. Regulating medicines in a globalized world: The need for increased reliance among regulators. Washington, DC: The National Academies Press.
NASEM. 2020b. Stronger food and drug regulatory systems abroad. Washington, DC: The National Academies Press.
NASEM. 2021. Ensuring an effective public health emergency medical countermeasures enterprise. Washington, DC: The National Academies Press.
NASEM. 2022a. Building resilience into the nation’s medical product supply chains. Washington, DC: The National Academies Press.
NASEM. 2022b. Globally resilient supply chains for seasonal and pandemic influenza vaccines. Washington, DC: The National Academies Press.
NASEM. 2023. Future of the nation’s laboratory systems for health emergency response: Proceedings of a workshop—in brief. Washington, DC: The National Academies Press.
National Academy for State Health Policy. 2024. State efforts to limit or enforce COVID-19 vaccine mandates. https://nashp.org/state-tracker/state-efforts-to-ban-or-enforce-covid-19-vaccine-mandates-and-passports (accessed February 7, 2024).
Ndodo, N., J. Ashcroft, K. Lewandowski, A. Yinka-Ogunleye, C. Chukwu, A. Ahmad, D. King, A. Akinpelu, C. Maluquer de Motes, P. Ribeca, R. P. Sumner, A. Rambaut, M. Chester, T. Maishman, O. Bamidele, N. Mba, O. Babatunde, O. Aruna, S. T. Pullan, B. Gannon, C. S. Brown, C. Ihekweazu, I. Adetifa, and D. O. Ulaeto. 2023. Distinct monkeypox virus lineages co-circulating in humans before 2022. Nature Medicine 29(9):2317–2324.
Nguyen, E., M. Poli, M. G. Durrant, A. W. Thomas, B. Kang, J. Sullivan, M. Y. Ng, A. Lewis, A. Patel, A. Lou, S. Ermon, S. A. Baccus, T. Hernandez-Boussard, C. Ré, P. D. Hsu, and B. L. Hie. 2024. Sequence modeling and design from molecular to genome scale with Evo. bioRxiv 2024.2002.2027.582234.
Ninove, L., Y. Domart, C. Vervel, C. Voinot, N. Salez, D. Raoult, H. Meyer, I. Capek, C. Zandotti, and R. N. Charrel. 2009. Cowpox virus transmission from pet rats to humans, France. Emerging Infectious Diseases 15(5):781–784.
Nou, X. A., and C. A. Voigt. 2024. Sentinel cells programmed to respond to environmental DNA including human sequences. Nature Chemical Biology 20(2):211–220.
Noyce, R. S., S. Lederman, and D. H. Evans. 2018. Construction of an infectious horsepox virus vaccine from chemically synthesized DNA fragments. PLOS One 13(1):e0188453.
NTI (Nuclear Threat Initiative). 2024. New international biosecurity organization launched to safeguard bioscience. https://www.nti.org/news/new-international-biosecurity-organization-launched-to-safeguard-bioscience (accessed February 29, 2024).
O’Toole, Á., R. A. Neher, N. Ndodo, V. Borges, B. Gannon, J. P. Gomes, N. Groves, D. J. King, D. Maloney, P. Lemey, K. Lewandowski, N. Loman, R. Myers, I. F. Omah, M. A. Suchard, M. Worobey, M. Chand, C. Ihekweazu, D. Ulaeto, I. Adetifa, and A. Rambaut. 2023. APOBEC3 deaminase editing in mpox virus as evidence for sustained human transmission since at least 2016. Science 382(6670):595–600.
Parrino, J., and B. S. Graham. 2006. Smallpox vaccines: Past, present, and future. Journal of Allergy and Clinical Immunology 118(6):1320–1326.
Pastoret, P. P., and A. Vanderplasschen. 2003. Poxviruses as vaccine vectors. Comparative Immunology, Microbiology, & Infectious Diseases 26(5–6):343–355.
Peng, C., S. L. Haller, M. M. Rahman, G. McFadden, and S. Rothenburg. 2016. Myxoma virus M156 is a specific inhibitor of rabbit PKR but contains a loss-of-function mutation in Australian virus isolates. Proceedings of the National Academy of Sciences 113(14):3855–3860.
Perdiguero, B., P. Pérez, L. Marcos-Villar, G. Albericio, D. Astorgano, E. Álvarez, L. Sin, C. E. Gómez, J. García-Arriaza, and M. Esteban. 2023. Highly attenuated poxvirus-based vaccines against emerging viral diseases. Journal of Molecular Biology 435(15):168173.
Petersen, B. W., and I. K. Damon. 2015. 136: Other poxviruses that infect humans: Parapoxviruses (including orf virus), molluscum contagiosum, and yatapoxviruses. In J. E. Bennett, R. Dolin and M. J. Blaser (eds.), Mandell, Douglas, and Bennett’s principles and practice of infectious diseases (8th edition). Philadelphia: W.B. Saunders. Pp. 1703–1706.
Petersen, B. W., I. K. Damon, C. A. Pertowski, D. Meaney-Delman, J. T. Guarnizo, R. H. Beigi, K. M. Edwards, M. C. Fisher, S. E. Frey, R. Lynfield, and R. E. Willoughby. 2015. Clinical guidance for smallpox vaccine use in a postevent vaccination program. Morbidity and Mortality Weekly Report Recommendations and Reports 64(2):1–32.
Petersen, B. W., T. J. Harms, M. G. Reynolds, and L. H. Harrison. 2016. Use of vaccinia virus smallpox vaccine in laboratory and health care personnel at risk for occupational exposure to orthopoxviruses—Recommendations of the Advisory Committee on Immunization Practices (ACIP), 2015. Morbidity and Mortality Weekly Report 65(10):257–262.
Qin, D. 2019. Next-generation sequencing and its clinical application. Cancer Biology & Medicine 16(1):4–10.
Reardon, S. 2014. Infectious diseases: Smallpox watch. Nature 509(7498):22–24.
Reynolds, M. G., W. B. Davidson, A. T. Curns, C. S. Conover, G. Huhn, J. P. Davis, M. Wegner, D. R. Croft, A. Newman, N. N. Obiesie, G. R. Hansen, P. L. Hays, P. Pontones, B. Beard, R. Teclaw, J. F. Howell, Z. Braden, R. C. Holman, K. L. Karem, and I. K. Damon. 2007. Spectrum of infection and risk factors for human monkeypox, United States, 2003. Emerging Infectious Diseases 13(9):1332–1339.
Robinson, P. C., D. F. L. Liew, H. L. Tanner, J. R. Grainger, R. A. Dwek, R. B. Reisler, L. Steinman, M. Feldmann, L. P. Ho, T. Hussell, P. Moss, D. Richards, and N. Zitzmann. 2022. COVID-19 therapeutics: Challenges and directions for the future. Proceedings of the National Academy of Sciences 119(15):e2119893119.
Sandbrink, J. B. 2023 (unpublished). Artificial intelligence and biological misuse: Differentiating risks of language models and biological design tools. arXiv https://doi.org/10.48550/arXiv.2306.13952.
Satheshkumar, P. S., and I. K. Damon. 2021. Chapter 17: Poxviruses. In P. M. Howley and D. M. Knipe (eds.), Fields virology: DNA viruses, vol. 2 (7th edition). Philadelphia: Wolters Kluwer Health. Pp. 614–640.
Schad, F., and A. Thronicke. 2022. Real-world evidence—current developments and perspectives. International Journal of Environmental Research and Public Health 19(16):10159.
Serafini, G., B. Parmigiani, A. Amerio, A. Aguglia, L. Sher, and M. Amore. 2020. The psychological impact of COVID-19 on the mental health in the general population. QJM 113(8):531–537.
Shao, B. 2024. A long-context language model for the generation of bacteriophage genomes. bioRxiv 2023.2012.2018.572218.
Sinclair, C. 2023. About emergent. Presentation at Meeting 3 of the Committee on Current State of Research, Development, and Stockpiling of Smallpox MCMs of the National Academies. December 14. https://www.nationalacademies.org/event/41411_12-2023_meeting-3-of-the-committee-on-the-current-state-of-research-development-and-stockpiling-of-smallpox-medical-countermeasures (accessed February 18, 2024).
Sluzala, Z., and E. Haislmaier. 2022. Lessons from COVID-19: How policymakers should reform the regulation of clinical testing. https://www.heritage.org/public-health/report/lessons-covid-19-how-policymakers-should-reform-the-regulation-clinical/#_ftnref9 (accessed March 2, 2024).
Smith, G. L., C. T. O. Benfield, C. Maluquer de Motes, M. Mazzon, S. W. J. Ember, B. J. Ferguson, and R. P. Sumner. 2013. Vaccinia virus immune evasion: Mechanisms, virulence and immunogenicity. Journal of General Virology 94(11):2367–2392.
Srinivasan, P., and C. D. Smolke. 2020. Biosynthesis of medicinal tropane alkaloids in yeast. Nature 585(7826):614–619.
Taub, D. D., W. B. Ershler, M. Janowski, A. Artz, M. L. Key, J. McKelvey, D. Muller, B. Moss, L. Ferrucci, P. L. Duffey, and D. L. Longo. 2008. Immunity from smallpox vaccine persists for decades: A longitudinal study. American Journal of Medicine 121(12):1058–1064.
Thi Nhu Thao, T., F. Labroussaa, N. Ebert, P. V’Kovski, H. Stalder, J. Portmann, J. Kelly, S. Steiner, M. Holwerda, A. Kratzel, M. Gultom, K. Schmied, L. Laloli, L. Hüsser, M. Wider, S. Pfaender, D. Hirt, V. Cippà, S. Crespo-Pomar, S. Schröder, D. Muth, D. Niemeyer, V. M. Corman, M. A. Müller, C. Drosten, R. Dijkman, J. Jores, and V. Thiel. 2020. Rapid reconstruction of SARS-CoV-2 using a synthetic genomics platform. Nature 582(7813):561–565.
Torres-Domínguez, L. E., and G. McFadden. 2019. Poxvirus oncolytic virotherapy. Expert Opinion on Biological Therapy 19(6):561–573.
Tucker, J. B. 2002. Scourge: The once and future threat of smallpox. Grove Press.
Upton, C., S. Slack, A. L. Hunter, A. Ehlers, and R. L. Roper. 2003. Poxvirus orthologous clusters: Toward defining the minimum essential poxvirus genome. Journal of Virology 77(13):7590–7600.
Van Dijck, C., N. A. Hoff, P. Mbala-Kingebeni, N. Low, M. Cevik, A. W. Rimoin, J. Kindrachuk, and L. Liesenborghs. 2023. Emergence of mpox in the post-smallpox era—A narrative review on mpox epidemiology. Clinical Microbiology and Infection 29(12):1487–1492.
Warfel, K. F., A. Williams, D. A. Wong, S. E. Sobol, P. Desai, J. Li, Y. F. Chang, M. P. DeLisa, A. S. Karim, and M. C. Jewett. 2023. A low-cost, thermostable, cell-free protein synthesis platform for on-demand production of conjugate vaccines. ACS Synthetic Biology 12(1):95–107.
White House. 2023. Executive order on the safe, secure, and trustworthy development and use of artificial intelligence, October 30, 2023. https://www.whitehouse.gov/briefing-room/presidential-actions/2023/10/30/executive-order-on-the-safe-secure-and-trustworthy-development-and-use-of-artificial-intelligence (accessed February 29, 2024).
WHO (World Health Organization). 2015. The Independent Advisory Group on Public Health Implications of Synthetic Biology Technology Related to Smallpox, June 2015. https://www.who.int/publications/i/item/the-independent-advisory-group-on-public-health-implications-of-synthetic-biology-technology-related-to-smallpox (accessed February 15, 2024).
WHO. 2022. Understanding the behavioural and social drivers of vaccine uptake: WHO position paper, May 2022. Weekly Epidemiological Record 97:209–224.
WHO. 2023a. Mpox (monkeypox)—Democratic Republic of the Congo. November 23. https://www.who.int/emergencies/disease-outbreak-news/item/2023-DON493 (accessed December 22, 2023).
WHO. 2023b. Multi-country outbreak of mpox, external situation report #31—22 December. https://www.who.int/publications/m/item/multi-country-outbreak-of-mpox--external-situation-report-31---22-december-2023 (accessed February 15, 2024).
WHO. 2023c. WHO Advisory Committee on Variola Virus Research: Report of the twenty-fourth meeting, Geneva, 29–30 November 2022. https://www.who.int/publications/i/item/9789240076310 (accessed February 28, 2024).
WHO. 2024a. 154th session of the executive board, provisional agenda item 18—Smallpox eradication: Destruction of variola virus stocks (January 2). https://apps.who.int/gb/ebwha/pdf_files/EB154/B154_20-en.pdf (accessed February 15, 2024).
WHO. 2024b. Variola virus repository safety inspections. https://www.who.int/activities/variola-virus-repository-safety-inspections (accessed February 4, 2024).
WHO Executive Board. 2024. Intervention by the delegate of the Russian Federation. 154th Session, Agenda Item 18. January 25. https://www.who.int/about/accountability/governance/executive-board/executive-board-154th-session (accessed February 28, 2024).
Yezli, S., and J. A. Otter. 2011. Minimum infective dose of the major human respiratory and enteric viruses transmitted through food and the environment. Food and Environmental Virology 3(1):1–30.
Yih, W. K., T. A. Lieu, V. H. Rêgo, M. A. O’Brien, D. K. Shay, D. S. Yokoe, and R. Platt. 2003. Attitudes of healthcare workers in U.S. hospitals regarding smallpox vaccination. BMC Public Health 3:20.
Yinka-Ogunleye, A., O. Aruna, D. Ogoina, N. Aworabhi, W. Eteng, S. Badaru, A. Mohammed, J. Agenyi, E. N. Etebu, T.-W. Numbere, A. Ndoreraho, E. Nkunzimana, Y. Disu, M. Dalhat, P. Nguku, A. Mohammed, M. Saleh, A. McCollum, K. Wilkins, O. Faye, A. Sall, C. Happi, N. Mba, O. Ojo, and C. Ihekweazu. 2018. Reemergence of human monkeypox in Nigeria, 2017. Emerging Infectious Diseases 24(6):1149–1151.
Yuan, S., H. Chu, J. F.-W. Chan, Z.-W. Ye, L. Wen, B. Yan, P.-M. Lai, K.-M. Tee, J. Huang, D. Chen, C. Li, X. Zhao, D. Yang, M. C. Chiu, C. Yip, V. K.-M. Poon, C. C.-S. Chan, K.-H. Sze, J. Zhou, I. H.-Y. Chan, K.-H. Kok, K. K.-W. To, R. Y.-T. Kao, J. Y.-N. Lau, D.-Y. Jin, S. Perlman, and K.-Y. Yuen. 2019. SREBP-dependent lipidomic reprogramming as a broad-spectrum antiviral target. Nature Communications 10(1):120.
Zhang, F., Q. Ji, J. Chaturvedi, M. Morales, Y. Mao, X. Meng, L. Dong, J. Deng, S. B. Qian, and Y. Xiang. 2023. Human SAMD9 is a poxvirus-activatable anticodon nuclease inhibiting codon-specific protein synthesis. Science Advances 9(23):eadh8502.
Zubair, M., J. Wang, Y. Yu, M. Faisal, M. Qi, A. U. Shah, Z. Feng, G. Shao, Y. Wang, and Q. Xiong. 2022. Proteomics approaches: A review regarding an importance of proteome analyses in understanding the pathogens and diseases. Frontiers in Veterinary Science 9:1079359.
This page intentionally left blank.