1
Ultra-Strong Molecules
BALLISTIC PERFORMANCE OF ULTRA-HIGH MOLECULAR WEIGHT POLYETHYLENE COMPOSITES
Vikram Deshpande, Professor, University of Cambridge
Vikram Deshpande opened his presentation by explaining that ultra-high molecular weight polyethylene consists of a carbon backbone with two hydrogen atoms for each carbon atom, as shown in Figure 1.1. While the carbon-carbon bonds are stiff and very strong, the hydrogen-hydrogen bonds between the molecules
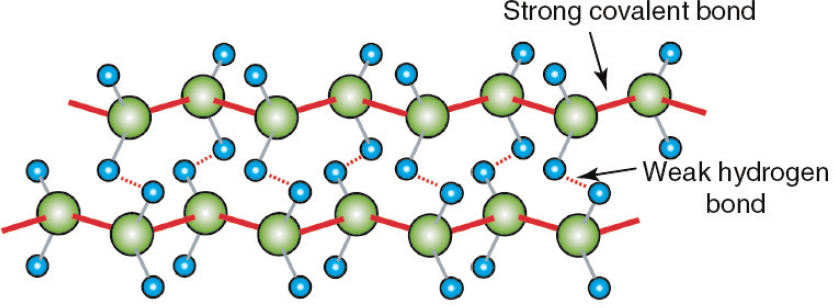
are much weaker due to van der Waals interactions. The high molecular weight molecules align along the axis of a fiber or in one direction in a tape, and great strength results from the high number of hydrogen bonds, perfect alignment, and low adhesion between these molecules.
Two failure mechanisms exist for such a perfectly aligned system, according to Deshpande: carbon bonds can break, and weak van der Waals bonds between molecules can shear (e.g., as the free end of a molecule is withdrawn from surrounding molecules). The former is rare for current length molecules because the ideal bond strength of the carbon-carbon bonds is on the order of 30 GPa, and so the shear mode occurs more often. When the molecules slide past each other during bond shear, the fiber strength decreases as the length of the molecules decreases. To maximize strength and avoid the intermolecular shear failure, he emphasized that the molecules need to be very long (greater than approximately 6 microns in length). Deshpande noted that DSM and Honeywell have made molecules on the order of 10 microns and assembled them into fibers. However, such commercial fibers rarely exceed a strength of 3 GPa, which is a lower than expected value. There are a number of possible reasons for the apparent low strength of the carbon-carbon bonds. They include the presence of methyl side groups on polyethylene molecule chains, poor alignment of the polyethylene chains, and dispersion of polyethylene molecule chain lengths. Deshpande pointed out that, although this strength may seem low, such a fiber is still exceptional because its density of 0.98 kg/m3 allows it to float on water. Two commercial fibers with strengths of approximately 3 GPa currently exist—Spectra and Dyneema,1 the Dutch equivalent of Spectra polyethylene fibers. He explained that while there are other fibers that have been developed with higher specific strength, those fibers used molecules that are not environmentally stable and are no longer commercially available. Deshpande discussed how ultra-high molecular weight polyethylene fibers and composites perform in a ballistics setting. He noted that ballistic protection is currently one of the largest applications of ultra-high molecular weight polyethylene composites because these materials usually have more than twice the ballistic limit of similarly weighted steel. To appreciate this strength, Deshpande explained, it is important to understand how the composite is made. Initially, a fiber is made using a process to pull out the high molecular weight polyethylene into an extended chain (crystalline) configuration. The fibers are then aligned in plies, and the plies are configured in a 90-degree orientation (see Figure 1.2). A small volume fraction (10-20 percent) of a thermoplastic resin is added to the plies, and the system is then compressed at a temperature just below that for melting of the polyethylene, forming a polymeric composite. When the fibers are stacked and consolidated this way, they contain a
___________________
1 Spectra is a brand name product made by Honeywell. Dyneema is a brand name product made by DSM.
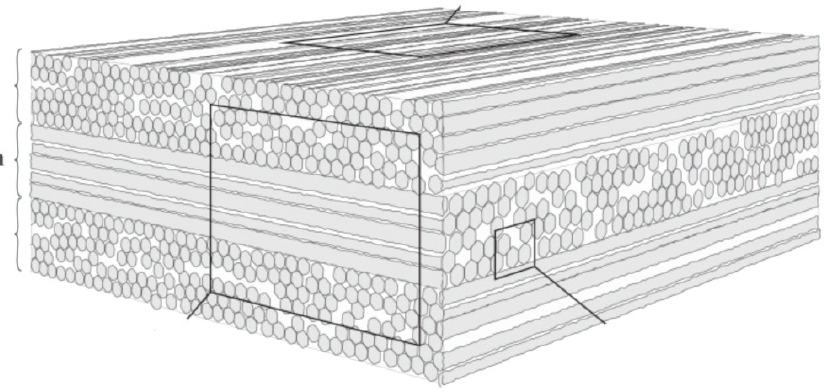
high-volume fraction (80-90 percent) of fibers, which increases the overall strength of the composite. A workshop participant asked how deformation at high strain rates influences the properties of these fibers. Deshpande explained that there are viscoelastic effects at low strain rates enabling gradual (creep-like) straining, but at high strain rates there is no time for molecular relaxations, and the fibers exhibit a strain rate sensitivity.
Deshpande introduced the 1996 hypothesis of Phil Cunniff, Army Research Laboratory, regarding the material metrics that determine the ballistic performance of fibrous systems. Cunniff identified a material group (the energy absorption per unit mass) and multiplied it by the elastic wave speed of the fiber. This number’s cube root has dimensions of velocity and is related to the velocity at which a bullet passes through the fibrous system. The precise velocity of penetration is a function of the mass of the fibrous plate and that of the mass of the projectile. When this is accounted for, it is possible to compare the ballistic resistance of fibrous systems. Deshpande noted that this material metric indicates that current grades of Dyneema or Spectra offer a better ballistic performance than current grades of Kevlar. Deshpande noted, however, that improving the material metric is only the first step. A beam experiment that illuminates what is happening beneath the projectile shows a progressive mode of failure (i.e., penetration) that is not captured in Cunniff’s model. His beam compression tests showed that the failure is related to the microstructure of the [0/90] ply structure within the composite. When a blunt projectile compresses a ply, it can undergo a very large lateral (Poisson’s ratio) displacement in the plane of the ply but at right angles to the fibers. This large displacement is in the direction of the fibers in the ply below the deforming
one, and this loads the fibers in tension by a shear lag mechanism. Eventually, the (indirect) tensile stress in the fibers causes their fracture and the projectile advances into the composite. The process then repeats until either the projectile is arrested or the sample is penetrated. This suggests that the fiber is not the only factor governing the strength of the composite (e.g., the ply arrangement is another consideration). Cunniff showed that the compressive strength is improved by increasing shear strength and decreasing ply thickness at constant fiber strength. In addition to increasing fiber strength or ply thickness, Deshpande explained, there are other ways to improve the ballistic performance of polyethylene-type composites. One approach is to alter the microstructure of the composite from one based on fibers to one based on tapes in which molecules are aligned in one direction within the plane of the tape (see Figure 1.3).
Tape-based composites offer an advantage in that they reduce the lateral displacement transverse to the molecule direction that is responsible for (indirect tension) failure. In other words, when tapes are used, the reduction in indirect tension should lead to improved ballistic performance. However, the tapes made by early fabrication processes have a lower ballistic limit than the fibers made from the same molecular weight polyethylene because of their poor molecular alignment. If the fibers with already aligned molecules are rolled to make tapes instead, performance improves.
Deshpande emphasized that not all ballistics issues involve blunt projectiles; some relate to sharp projectiles. There is also growing interest in protecting law-enforcement officers from knife wounds. A Mode I crack results when a knife
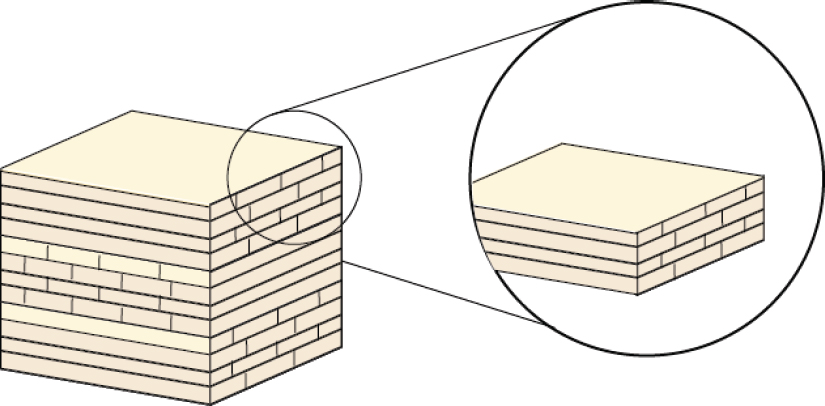
enters a polyethylene composite, causing the fibers to split apart. Because of the ply structure of the composite, the cracks will be different in each ply layer, creating additional Mode II cracks. Deshpande noted that this process causes kinetic energy of a sharp penetrator to be dissipated and that the microstructure could be modified to improve the performance. In other words, the ply could be thinner to generate more cracks and increase the penetration pressure, ultimately offering better protection (see Figure 1.4).
Peter Liaw, University of Tennessee, asked if the wiggles in the curve in Figure 1.4 were due to noise. Deshpande said that the wiggles corresponded to interfacial fracture, not to noise. Liaw then proposed analyzing those results using statistical physics. Karin Dahmen, University of Illinois, Urbana-Champaign, noted that histograms of the size of the wiggles might provide interesting information. Deshpande agreed that this would be a worthy exercise to gather information about
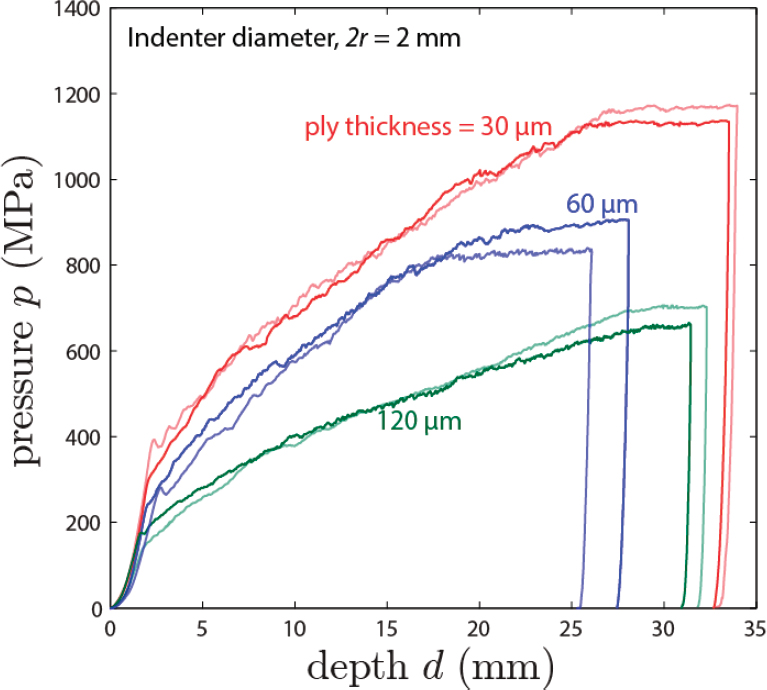
the fracture process and location. Liaw referred back to the causes of the wiggles in the curve at the highest strain rate and noted that the wiggles grow larger with decreased ply thickness. Deshpande said that they are due to the failure mechanism of the slipping of the molecules, and he also noted that it is difficult to measure the force dynamically in such conditions. Deshpande emphasized that understanding the mechanics of materials is crucial to advancing the science of ballistic systems. Noam Bernstein, Naval Research Laboratory, asked which material properties relate to Mode II fracturing. Deshpande noted that in order to reduce Mode II fracturing, the modulus of the fibers would need to be reduced to decrease the loading on the interfaces. He clarified that this is not a good idea because it results in the loss of the mechanism where the fibers have to bend around the indent, causing the penetration pressure to decrease.
Deshpande turned to a discussion of anisotropic materials, noting that one-dimensional (1D) materials can have substantially better properties because all of the molecules are aligned in a single direction. Graphene sheets have the potential to be remarkably strong, he noted, but it remains to be seen whether it is possible to make them at a suitable scale for ballistic functions. Deshpande pointed to work from Hernandez et al. (2008), who have experimented with creating composites of graphene but have not achieved perfect alignment or size of the graphene flakes. Deshpande noted that these are the same issues as those for polyethylene molecules, so the general mechanics of different types of composites are not that different from one another. He pointed out that graphene composites have the potential to make worthy ballistic materials in the future; however, they are unlikely to serve a structural role because, like polyethylene composites, their shear strength is too low.
Deshpande reiterated two key assertions about the improvement of the ballistic performance of ultra-high molecular weight polyethylene and other high-performance fiber composites: (1) the properties of the parent materials need to be enhanced (e.g., create better fibers with higher molecular weights and better alignments or explore other two-dimensional [2D] materials); and (2) the composites need to experience microstructural improvements (e.g., use tapes, decrease ply thickness, or create matrix systems that provide higher interfacial toughness).
Ward Plummer, Louisiana State University, asked about the reproducibility of Deshpande’s measurements and findings. Deshpande reported that data for commercially available fiber (2.5 GPa) are extremely repeatable. However, there is more scatter in the data with the composite based upon research-grade materials, so noncommercially available, higher-strength fibers are less reproducible. Daniel Miracle, Air Force Research Laboratory, asked if the role of defects in controlling the material properties (e.g., defects in the polymer or the interface due to processing) had been examined. Deshpande agreed that this topic is worthy of discussion. He noted that the defects at the composite level are significant. Rosario Gerhardt, Georgia Institute of Technology, asked Deshpande if he had a standard deviation
to confirm his claims about penetration mechanisms. Deshpande agreed with Gerhardt that more data are needed to make these assertions more statistically meaningful.
WHAT LIMITS THE STRENGTH OF FIBERS? MOLECULES, PROCESSING, . . . ?
Satish Kumar, Professor, Georgia Institute of Technology
Satish Kumar opened his presentation noting that although removing polymer molecular entanglements and defects is not an easy process, it is crucial in order to increase strength. He introduced various types of strong fibers and their theoretical strengths: Zylon (30 GPa), Kevlar (30 GPa), Spectra and Dyneema (30 GPa), and IM7 and K1100 (100 GPa). The K1100 fiber has been produced commercially, but the production of this fiber was discontinued because the market for this carbon fiber was too small. Kumar noted that continuous-length fibers have been commercially produced with a modulus of up to approximately 91 percent of the theoretical value, although the tensile strength of these fibers will only be 5 to 15 percent of the theoretical value.
Kumar recalled the man-made fibers of the 1930s and 1940s that had many amorphous regions. Although these regions remain in materials like polyester and nylon, by 1980 these amorphous regions had been eliminated with the development of some new materials such as polyethylene in the form of Spectra and Dyneema. Removing amorphous regions resulted in an improvement in tensile strength by a factor of 10. However, even with the removal of amorphous regions, the following defects still exist in the new materials: voids, chain entanglements, chain bends, and chain ends. Since the ultimate goal is to create a material with the greatest amount of strength, further work needs to be done to eliminate voids and entanglements from the fiber structure (see Figure 1.5).
Kumar discussed the importance of understanding the basic structure of carbon fibers in order to try to eliminate the defects. He noted that although carbon fibers used to be made from cellulose, most carbon fibers in use today are made from polyacrylonitrile, which is a synthetic, semicrystalline organic polymer resin that balances tensile strength, compressive strength, and tensile modulus. Kumar noted, however, that polyacrylonitrile-based carbon fiber does not have the highest electrical or thermal conductivity. Maximum tensile strength of polyacrylonitrile currently occurs at a carbonization temperature of about 1,400°C, although the fiber can be graphitized to 2,800°C for specialized applications that require lower strength. In 2010, after approximately 50 years of research and development, these fibers were used in a commercial aircraft in significant quantities (e.g., Boeing 787). However, there are many production challenges for mass-scale usage of these
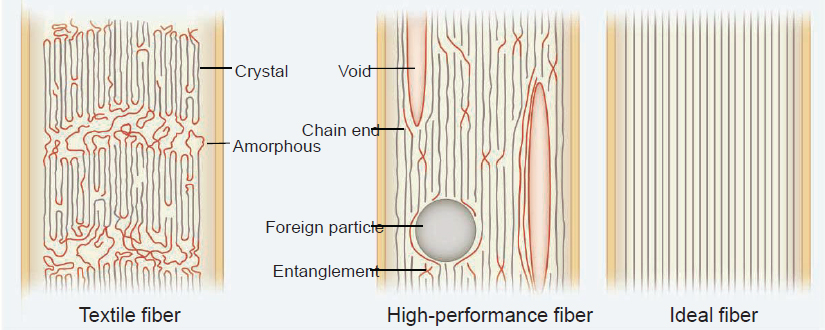
carbon fibers, such as those in the automobile industry. These include carbon fiber production capacity and cost as well as the time required for manufacturing composite parts.
Kumar returned to his discussion on defects with an emphasis on eliminating entanglements to double the strength of the fiber. Polyethylene fibers produced using the gel spinning process have a solution with 5 to 15 percent concentration. This can lead to many entanglements that will cause defects in the final material. Decreasing the concentration by half can reduce the entanglements substantially. He explained that it is possible to make carbon fibers that have the potential to increase both electrical and thermal conductivity; for example, one can use carbon nanotubes in polyacrylonitrile.
In the last 35 years, Kumar and his team have continued to make high-strength carbon and polymeric fibers. Over the past 5 years, Kumar’s team has achieved a 30 percent higher modulus at the same strength with fewer entanglements. He noted that these high-strength, high-modulus fibers have been produced only in a 100-filament tow, but that strength will continue to increase with further increases in the number of filaments in the tow. In showing a series of cross sections, Kumar highlighted the inhomogeneity of different regions of material (e.g., amorphous, crystalline)—for example, the IM7 carbon fiber is not as perfect a structure as that achieved through the gel spinning process. Kumar also noted the difference between high-strength carbon fibers, which have an intergraphitic plane shear modulus of approximately 30 GPa, and graphite and graphene, which have an interplanar shear modulus of approximately 4 GPa. In response to a question from Haydn Wadley, University of Virginia, Kumar acknowledged that the “perfect” carbon fiber would have a strength of over 100 GPa with a modulus of 1,060 GPa.
There are certain graphene properties that can be achieved in carbon fiber, but it is currently impossible to achieve similar tensile or compressive strength; perfect carbon nanotubes produced in a bottom-up process better imitate the desired properties, according to Kumar.
Kumar noted that if it is possible to make hollow carbon fibers, carbon fiber composites could foreseeably be made with a density less than water, allowing airplanes to land on water; Boeing is currently funding research on hollow carbon fibers. Other advantages of these hollow carbon fibers with continuous carbonization include higher alignment and higher modulus, as well as reduced wall thickness. Kumar hypothesized that with the reduction in wall thickness, the gases that are released will have a shorter distance to travel, thus decreasing the opportunity for structural defects and increasing overall strength.
Peter Liaw asked Kumar if corrosion is a concern for the carbon nanotube fiber. Kumar responded that he is unaware of this being an issue. Daniel Miracle agreed that it would likely not be an issue unless the corrosion occurred in hot oxygen. Luigi Colombo, Texas Instruments, Inc., asked Kumar to elaborate on the nature of the defects he uncovered. Kumar noted that the defects are point, volumetric, and 2D. Colombo then asked if anyone has carefully compared graphite and polycrystalline graphite in terms of strength and ballistic performance. Kumar responded that this is something he would like to explore in greater detail. Steven Fishman, retired from the Office of Naval Research, asked about the resulting effects of including carbon nanotubes, which have weak bonding, in carbon fibers. Kumar explained that each end of the nanotube becomes a defect site, which creates a negative effect on tensile strength. If tubes can be made long enough to decrease the frequency of defects, the positive effects of strength would be apparent. Ward Plummer asked Kumar to respond to concerns about process management and reproducibility. Kumar said that process challenges exist and provided an example of an experimental fiber (GT-PAN) with similar parameters but different ways of dissolving the polymer powder. He noted that because this is such a small process detail, it is often not noted in scientific publications, which leads to the difficulty in reproducibility (e.g., even a detail this small creates a difference in dissolution time of several hours). Kumar noted that his team has identified nearly 100 different factors related to problems in processing, including the size of the polymer particle.
A workshop participant asked if there are ways to reduce the high costs and the complicated process associated with making carbon fibers, while still achieving the same strength. Kumar remarked that there are three things that contribute nearly equally to the cost of carbon fiber: the cost of raw material, the cost of energy, and the cost of the infrastructure. He also noted that too much heat is wasted during the production of carbon fiber, so current efforts are dedicated to reducing the cost of energy—for example, carbon nanotubes distributed throughout the fiber can be used as Joule heating elements to assist in stabilizing and possibly carbonizing the
fiber when electric current is applied. This innovation has the potential to reduce energy use by 50 to 90 percent, which could ultimately reduce the cost by 15 to 25 percent. Last, using a renewable raw material such as lignin also has the potential to reduce the cost of carbon fiber. However, lignin-based carbon fibers currently have relatively low tensile strength.
MECHANICS OF GRAPHENE
James Hone, Wang Fong-Jen Professor, Columbia University
James Hone began his presentation with a short history on the evolution of graphene. Twenty years ago, he noted, researchers looked at carbon allotropes such as fullerenes, which led to a study of nanotubes, which ultimately led to the study of graphene. The first major breakthrough in graphene research occurred when Novoselov et al. (2004), University of Manchester, demonstrated the possibility of isolating thin layers of carbon using the “tape method.” The tape method is a standard technique (predating this experiment) for cleaving layered crystals and placing the debris field on a wafer. Novoselov et al. were then able to image ultrathin layers using an optical microscope to sort through the debris field and find monolayers or bilayers. This was the first experimental extraction of graphene.
Hone pointed out that graphene flakes could also be suspended over holes etched into wafers, instead of just placed on a flat substrate. After indenting the center with atomic force microscopy (AFM) during a mechanical test of the graphene, he noted the following two advantages: (1) symmetry allows for easier modeling because the problem has become 1D, and (2) high-stress concentration at the center and low-stress concentration on the edge upon breaking shows that the van der Waals adhesion is enough to avoid using additional clamps on the graphene membrane, thereby eliminating stress at the clamp sites. This is significant given that mechanical testing on a carbon nanotube, another 1D system, traditionally fails at the clamp sites, he explained.
Hone described a scanning electron microscopy-based nanoindentation test where an AFM or a nanoindentation system is used to find the center of the graphene membrane and indentation. After a large deflection for a 1-atom thick material, the membrane broke. The emerging data indicate that the representative force-displacement response can be charted to demonstrate a semi-empirical relationship. As the area of the membrane increases from the indentation, the mechanical stiffness changes, Hone explained. The force has a linear and cubic form of displacement. While this is a nonlinear equation, the nonlinearity is only due to geometry. The linear term shows initial tension in the membrane (how the tape was rubbed with the tweezers), and the cubic term shows Young’s modulus, which is approximately 340 N/m. If this value is divided by the interlayer
spacing in graphite, a three-dimensional (3D) in-place Young’s modulus results (approximately 1 terapascal [TPa]), which is expected for a monolayer of graphene. Those who have claimed larger values (such as 2-7 TPa) have not made reasonable arguments, according to Hone, because it is impossible for the sheets to become stiffer to that extent through exfoliation.
Hone discussed the connection between measuring the breaking force and extracting the breaking strength of graphene. His team observed that the breaking load has no relation to membrane size because the local strain around the indenter tip is the only important factor; the radius of the tip curvature is the element that determines the breaking load. His team also discovered that silicon AFM tips broke when going through only one atomic layer of carbon, so they shifted to stronger diamond tips and measured the radius in transmission electron microscopy (TEM) both before and after the experiment. The next step after measuring the breaking load was to measure the strength from the breaking force. Hone clarified that although it is possible to find linear elasticity formulas for this, such formulas do not usually give good answers: the deduced strength is too high, and the strain limit indicated by finite element modeling is greater than the linear regime. The solution to this problem is a nonlinear elasticity equation. The breaking force showed Gaussian statistics instead of Weibull statistics, indicating that the observed fracture was not random or from defects; the membrane was breaking at its intrinsic strength (i.e., the point at which the stress-strain curve shows a maximum). Knowing this, Hone continued, one can adjust the nonlinear elastic parameter such that the finite element stimulation matches the measure breaking force. In the experiment itself, failure happens right underneath the center of the tip, although fracture occurs only if the supporting ring moves out of the radius of the indenter tip. After this experiment, Hone and his team proposed that graphene has strength equivalent to 130 GPa, a strength higher than that of any other material (see Figure 1.6).
Because Hone and his team were concerned that they had oversimplified the problem, they carried out a more rigorous analysis by expanding the elastic energy to the fifth order and differentiating to obtain a tensor equation for the stress-strain response. These tensors contain thousands of terms, but the high symmetry of graphene can be used to show that there are only 14 independent nonlinear elastic constants. However, only two of the linear elastic parameters are well understood. Hone noted that instead of attempting an experiment at this point to understand the other parameters, they used ab initio theory, ran in silico experiments on the computer, and stretched the graphene in different ways to generate data on the relationship between stress and strain. These simulations allowed the team to estimate all 14 elastic constants. The next step, Hone explained, was to use the estimates for these parameters in ABAQUS finite element simulations to create a multiscale model that could be used to compare experiments and to model different tip radii. Through the nonlinear terms, it is possible to observe anisotropy, reflecting the
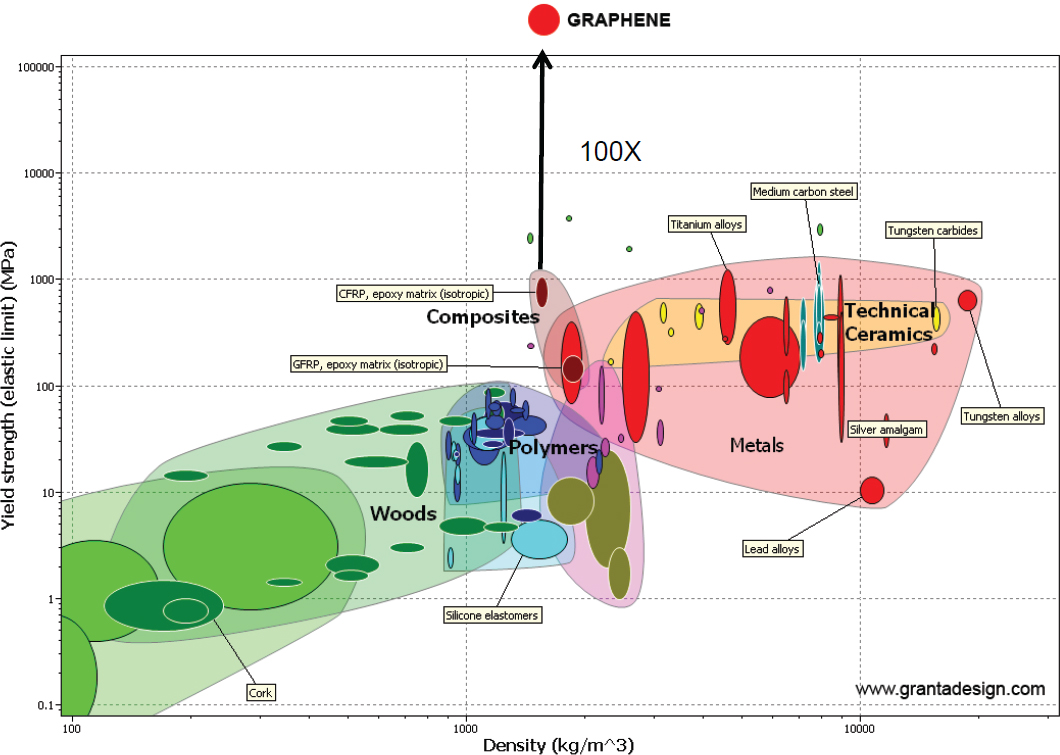
six-fold symmetry of the underlying crystal lattice, and it is possible to plot stress versus radial position. Hone continued that it is possible to more carefully fit the force displacement curves, but more importantly, it is possible to plot stress versus applied load using these different models. Stress remains constant in the fifth-order model even as the load increases. Through this improved nonlinear modeling, Hone observed that the strongest graphene is actually 100 GPa, not the previously thought 130 GPa.
Beyond understanding pristine graphene, Hone suggested, this model can be used to better understand how defects (both grain boundaries and point defects) impact the characteristics (especially the strength) of graphene. Chemical vapor deposition (CVD) is used in the laboratory to grow graphene with grain boundaries that can be viewed with TEM. The impetus for this work was that while previous experiments suggested CVD graphene would be weak, the theory suggested it would be quite strong. As part of this study, Hone’s team used an industrial nanoindenter for the membranes in order to collect better statistics and measurements than those collected with AFM. Using this nanoindenter, the team confirmed its hypothesis that grain boundaries do not affect stiffness. Ultimately, the team was able to do the indentation test directly on the grain boundary to study the change in loading force. The downward shift in breaking load for small-grain graphene from pristine large-grain graphene resulted in only an approximately 5 percent reduction in strength, which confirms that on this particular scale CVD graphene is nearly as strong as intrinsic graphene.
Hone highlighted another experiment in which he and his team created point defects with O2 plasma, which initially adds atomic oxygen bonded to the graphene and then begins to remove carbon to create vacancies. Oxygen bonding decreases the stiffness monotonically but results in only a 14 percent reduction in strength, whereas vacancies decrease the strength more substantially. Hone clarified that although there is more work to do, this is a starting point in understanding guidelines for designing layered composites. In response to a participant’s question, Hone noted that it is possible to measure the lengths between defects as a means to understand defect density, which, in this case, is approximately one defect every 4 nanometers.
Current issues of interest for Hone are how strength will scale at the macroscopic level and how statistical modeling of failure at different scales can be useful. His objective is to put grain boundary information from both experiments and models into finite element simulations using the cohesive zone model. Hone plans to pursue composite materials: although graphene shows promise as a filler in nanocomposites, it is expensive. Hone is also interested in the study of flexible electronics and freestanding membranes as applications of graphene.
Karin Dahmen asked Hone if he has evaluated the statistics of the noise that is produced when pushing on a grain boundary observed in the load versus
displacement curve. Hone responded that the wiggles in that curve indicate only instrument noise, because there is no hysteresis, and that all of the fracture recorded is brittle fracture. The 20-30 data points collected so far on predictions for the failure strength as a function of grain boundary tilt angle do not currently correspond to theory. One issue here is that the grain boundaries are not atomically straight, and the strength depends on locating the bond with the largest built-in strain, Hone said. Valerie Browning, ValTech Solutions, LLC, returned to the issue of reproducibility and asked Hone if the 20-30 grain samples closely relate to one another. He affirmed that they all fall roughly within the same band.
SCALABLE METAMATERIALS
Xiaoyu “Rayne” Zheng, Professor of Mechanical Engineering and Director, Advanced Manufacturing and Metamaterials, Virginia Polytechnic Institute and State University
Xiaoyu Zheng introduced his presentation with an overview of the manufacturing capabilities of scalable metamaterials. He noted that the production of 3D architected metamaterials is expanding because of additive manufacturing at both the macro-micro-length and nano-length scales. This gives rise to unique mechanical, electromagnetic, and optical properties, he explained.
Zheng noted that his talk would focus specifically on scalable mechanical metamaterials, which are materials with 3D microscale features that have novel properties (e.g., ultralight, ultrastiff and strong, tunable negative Poisson ratios, or negative thermal expansion). His objective is to tune mechanical and multifunctional properties through creating a large array of hierarchical architected unit cells. One type of mechanical metamaterial that Zheng discussed contains a collection of stretch-dominated unit cells. According to his research, additive fabrication technologies provide the freedom to fabricate 3D architectures of any arbitrary topology. Through a process called “projection micro/nano stereolithography,” a digital light additive manufacturing technique is used to fabricate the unit cells into metallic lattices or ceramic hollow tube lattices, which gives rise to their ultra-lightweight, strong, and ductile materials. The fabrication is not dependent on the complexity of the geometry and can be done quickly across multiple length scales. Zheng explained multiple adaptations of these additive manufacturing approaches, including capabilities of incorporating multiple materials as well as scaling up to more than seven orders of magnitude from tens of nanometers to tens of centimeters and above. As a result, these micro/nano lattice materials can support a weight of over 16,000 times their own weight, and they have near-linear scaling between the density and Young’s modulus. Zheng found that the
order-of-magnitude performance improvement and decoupling of mutually exclusive mechanical properties (i.e., strength, toughness, density, and stiffness) has been achieved with these hierarchical stretch-dominated micro-architectures.
These metamaterials can be converted to various feedstock materials for different applications, Zheng explained, via a variety of postprocessing techniques such as sintering and pyrolysis, autocatalytic depositions, and atomic later deposition. Using these techniques, the wall thickness and the relative density of the material are reduced, while the aspect ratio is increased. The strength of this material is governed by the shell and tube buckling. Zheng continued that there is a limit to the strength because the ratio of the wall thickness to the diameter determines whether it will buckle.
Ultimately, Zheng acknowledged, scalability resulting from existing micro/nano fabrication technology is highly limited because of the challenges of obtaining both high-resolution and large-scale parts, as well as the precise control of designed topologies, which means that such technologies are currently impractical for real-world applications. Zheng described his goal as pushing the outer limits of scalability by increasing the feature size to achieve a length scale span over six orders of magnitude, which already occurs in nature.
Zheng introduced a new technology that is used by his team called fast-scanning dynamic mask lithography. The unique features of this technology allow projection of the whole pattern, resulting in a better hierarchical span of scalability. To do this, large-scale features are scanned onto the polymer bath, and each scanned area speeds scalability. These structures can be manufactured in hours, and the resolution can be altered as needed. To study the mechanics of the hierarchical lattices with multiple orders of magnitude, Zheng explained, the structure is divided into multiple length scales. A micro-architecture can be deterministically inserted into each length scale, resulting in multiscale hierarchical metamaterials (see Figure 1.7). The hollow tube is the smallest, ranging in wall thickness up to one micron. Through multiscale architectures spanning a wide range of length scale, deformation mechanisms can be tuned and designed into each order of hierarchy, he noted.
All of these geometric factors can be scanned at multiple length scales. The goal, Zheng reiterated, is to find optimal material strength in compression, shear, and tension at a particular relative density. It is also possible at this point to shear test the structures, which highlights the two-buckling mode failure (see Figure 1.8).
Zheng’s group also studied the hollow-tube hybrid multiscale lattices and discovered that it is possible to achieve super elasticity in metallic metamaterials. Zheng’s group also used fabrication technology to study tensile behavior of bend-stretch lattices, discovering that thicker walls experience brittle failure, while thinner walls combined with multiscale architectures are more ductile and highly
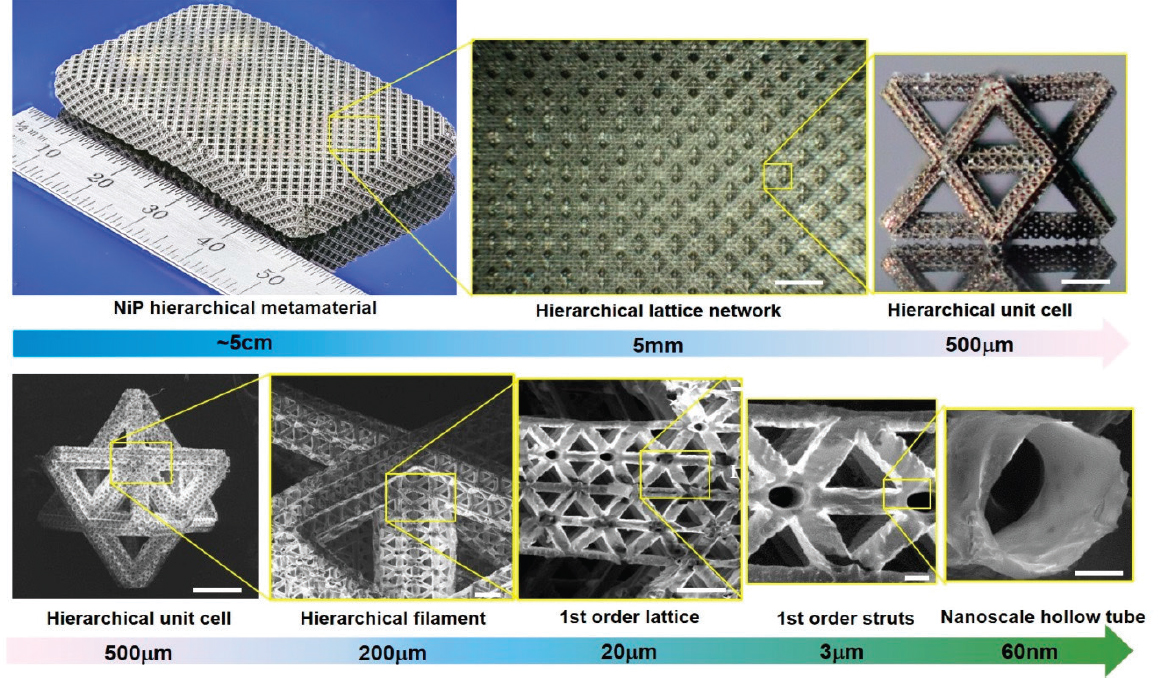
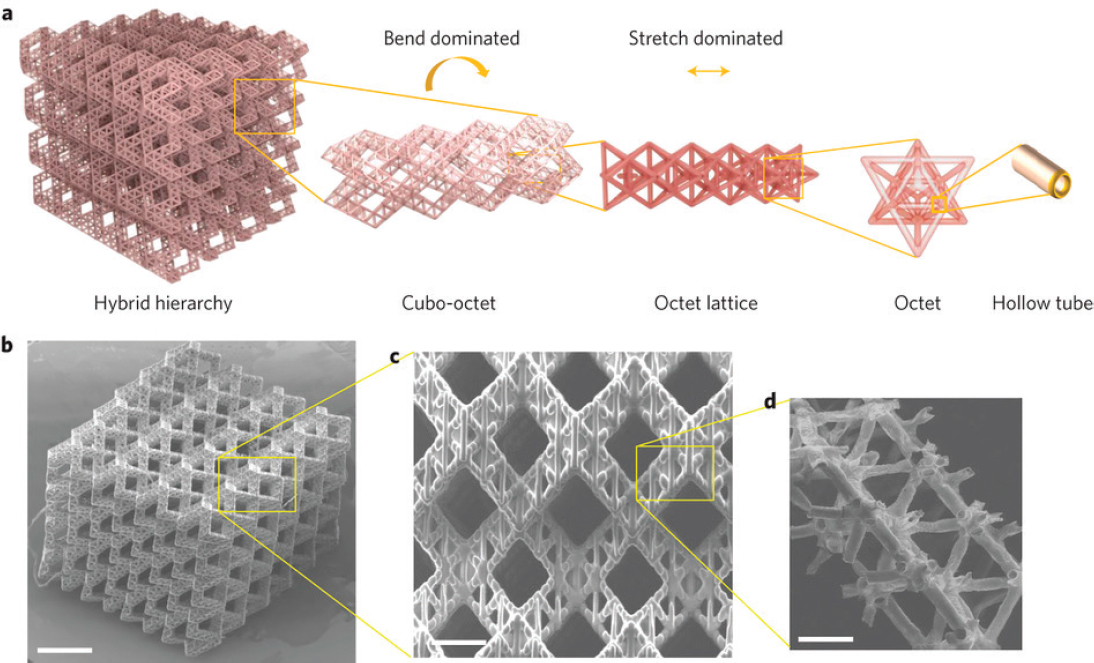
elastic. The stress of such a structure can be modeled, and the tensile strains versus specific strength have been plotted, outperforming the state-of-the-art lightweight metallic alloy structures with higher tensile strength with low density.
Last, Zheng noted that his group has also been working on creating ultrastrong, scalable metamaterials in high-temperature ceramic and carbon-based materials such as graphene, with controlled 3D topologies. These materials may find a variety of new applications ranging from thermal conduction or isolations, energy conversions, and storage devices.
Peter Liaw asked if high-entropy alloys (HEAs)2 could be used to fabricate this kind of structure. Zheng responded that this is possible through one of two processes: (1) consider how an HEA can be mixed with photopolymers to create a composite material, and then the two can be sintered together; and (2) deposit the HEAs into a scaffold and then etch off the polymer to create the hollow tube. Another workshop participant asked if it is possible to mix solid and hollow tubes to allow for different compression and tension characteristics. Zheng noted that they can all be modeled into the topologies and that his modeling approach allows for such flexibility.
___________________
2 High-entropy alloys are metals with usually five or more constituents in near equal quantities.