1
Introduction: Framing the Issues
The provision of electric power that is safe and secure, clean and sustainable, affordable and equitable, and reliable and resilient, is becoming ever more essential to social welfare and economic productivity in the United States.
Motivated by this fact, the congressional language that gave rise to this committee called for “an evaluation of the expected medium- and long-term evolution of the grid … [focused] on developments that include the emergence of new technologies, planning and operating techniques, grid architecture, and business models.” The committee’s statement of task is included in Appendix A.
Forecasting the likely evolution of the electric power system decades into the future is an extremely difficult problem because so much change is under way and so many currently uncertain developments will shape the way the system continues to evolve. Much as no single entity plans the Internet, no single entity is responsible for planning the future of the power system. The system is evolving in different ways in different parts of the country under the influence of many actors.
The evolution of the power system is shaped by very different pressures from those that shape the Internet, including a legacy of decades-old legal and regulatory requirements, some at the level of individual states, some at a regional level, and some at a national level. As noted in Chapter 3, in order to avoid uneconomic outcomes, stranded generation or transmission costs, and an erosion in reliability and resilience, there is a pressing need for flexible, adaptive, and credible planning. This is especially true in the context of regional or multiregional system operations and in accommodating possible future disruptive changes, especially at the grid edge.
For these and other reasons, this report does not tell readers how the nation’s electric system should evolve. Rather, it discusses a range of ways in which the power system might plausibly evolve. It also notes how current trends in characteristics such as the generation mix and technologies connected to the grid may influence its evolution. The report identifies a number of social, economic, regulatory, legal, political, technological, and engineering developments that could shape that evolution over the course of the next several decades. It discusses the impacts of those plausible developments—especially as they relate to considerations such as equity, reliability, resilience, environmental impact, consumer options, and cost. These considerations inform the series of core attributes, detailed in the following section, that this committee believes our electricity system must balance. Last, and perhaps most importantly, given the committee’s charge, the report identifies key research and policy choices that, if made today, could create options to enable a range of future developments.
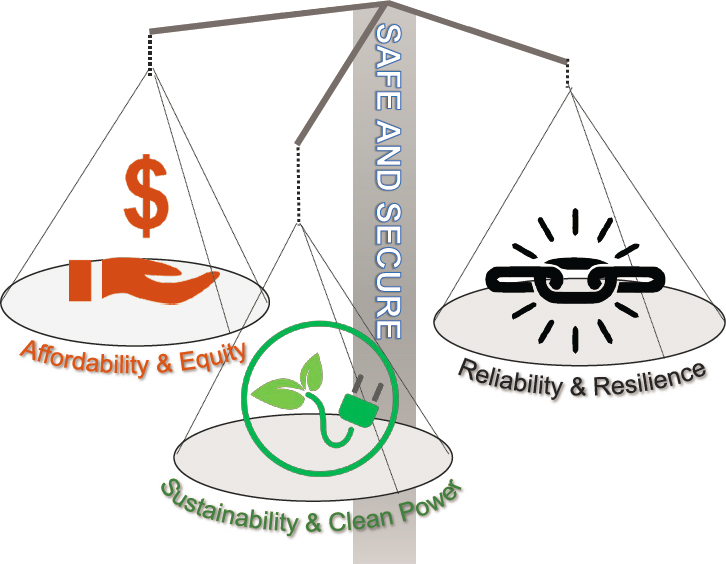
CORE ELECTRICITY SYSTEM ATTRIBUTES
Throughout this report, the committee stresses the importance of maintaining and strengthening an electric power system that is at its core safe and secure. Around that basic pillar, three other pairs of attributes must be balanced. These are that the electric system must be clean and sustainable, affordable and equitable, and reliable and resilient. These basic assumptions are illustrated in Figure 1.1.
-
Safe and secure: For decades, ensuring safety while keeping the lights on has been a bedrock objective for those who operate and regulate the electricity system. Further, making the grid and its components secure has also been central, for both its physical and cyber elements. The electricity industry must continue, and build upon, its outstanding record of past performance. This includes
- Developing better technologies and management strategies to ensure public and worker safety in the face of growing numbers and severity of extreme weather events such as wildfires, hurricanes, and ice storms, as well as geophysical events such as earthquakes and solar storms.
- Ensuring that continued attention to safe operation as a larger proportion of the functions that once fell in the domain of utility operators now fall in the domain of non-utility providers and customers.
- Increasing the use of automation and other technologies that are used to build and operate the system, without endangering the livelihood of utility employees.
- Clean and sustainable: The United States must accelerate the transition to a clean electricity system that produces no conventional air pollution; adds no net greenhouse gas emissions to the atmosphere; minimizes terrestrial and aquatic impacts; and imposes low ecosystem disruption. This must be done in ways that expand the system’s ability to generate and move power so as to make abundant electricity available to support the deep decarbonization of all parts of the economy. Advancing this objective will require
- Virtually eliminating the emission of conventional air pollution that today contributes to the premature deaths of tens of thousands of Americans.
- Developing and deploying a large and diverse portfolio of carbon-free generation technologies, particularly as electrification is pursued as a means to reduce dramatically, and ultimately eliminate, emissions from buildings, transportation, and industrial sectors, and improved technologies for storing and moving large quantities of energy across space and time.
- Minimizing adverse land and water use and ecological impacts from the power system.
- Adopting a longer-term perspective with respect to system planning and decision making on projects and systems to reduce the carbon intensity of the electricity system so as to avoid major near-term investment in capital stock, and the creation of institutions, which achieve short-term improvements, but make deep decarbonization harder and more expensive to achieve in the long run. Such choices in the near term could both slow needed transitions and give rise to large future stranded investments.
- Ensuring that the cost of electric service is affordable will continue to be a key to strong and equitable economic growth.
- Making concerted efforts to continue to balance the benefits and costs of system upgrades in a way that ensures that changes in investment and operational strategies result in an increase in net social benefits.
- Ensuring that affordable basic electric service is available to all Americans and reducing dramatically the level of energy poverty.
- Developing a secure system that can minimize the risks from physical and cyber disruptions and respond in a flexible and adaptive manner when disruptions occur.
- Acknowledging that because there is no way to make power systems completely invulnerable to intentional or accidental physical or cyber disruptions and to the effects of extreme weather events, the nation must move aggressively to create systems that can continue to provide basic services as they recover from disruptions.
- Implementing technologies and polices that provide high-quality and highly reliable power to sensitive digital loads without compromising the quality and cost of service that is provided to regular customers.
- Creating a regulatory and economic environment that increases the rate of innovation and its deployment at the level of distribution systems and customers (e.g., distributed generation and storage on both sides of the meter; systems for combined heat and power; microgrids with a variety of different ownership and operational arrangements).
Throughout this report, the committee advances findings and makes recommendations that are motivated by a continuing recognition of the key role of safe and secure operations while also needing to make continual tradeoffs among the other system attributes. One might ask, “Why not include safety as an additional attribute among trade-offs?” For example, electricity can cause injury and death, and there are inherent safety risks associated with all energy technologies. The committee’s answer is the same as the one Paul O’Neil gave when, as chief executive
officer of the Alcoa Corporation, he achieved a fundamental transformation in work-place safety: There are few core things about which one simply does not compromise.
Beyond that, the committee finds there will be a continuing need for
- A much larger political and economic commitment to the support of research and development across all elements of the energy system so that there is at least a tripling of the level of private and public investment in electricity and other energy research.
- The development of regulations, business models, and incentives that encourage innovation, and unleash the creative energies of many different parties.
- Finding ways to do improved holistic planning in a system in which many changes occur incrementally and are implemented by a wide variety of governmental and private actors across many different parts of the country.
- Finding ways to use the states as laboratories to experiment with, and learn how to improve, public regulation and policy, especially at the level of distribution systems. In parallel with doing this, developing strategies to extract, share, adapt, and implement the lessons learned in many other jurisdictions.
- A recognition that traditional systems of innovation and traditional supply chains for electric power systems are breaking down. Many new system innovations and technologies are being pioneered elsewhere in the world. The United States will need to adopt national policies, and promote international policies, that accommodate these changes, allowing the nation to efficiently learn and import from others.
FORECASTING THE FUTURE OF ELECTRIC POWER
What will the U.S. electric power system look like 10 or 30 years from now? No one knows, of course. On the one hand, because so much of the electric system consists of long-lived facilities and other capital stock, one can be reasonably confident that on the surface much of it will still physically look very much the same as it does today. Indeed, many of the same power plants that were in place 30 years ago are still in operation, nearly all of the same giant power lines and substations that span the country remain in the same physical location, and in most communities the poles and wires that bring power to homes and businesses look largely unchanged.
But physical appearances can be deceiving. A closer examination reveals that the electric system has undergone important changes in ownership and control, industry structures, generation technologies and costs, fuel prices, and public policies. Many of the changes evident today were not anticipated, even 10–15 years ago.
One can be very confident that profound changes will continue to occur over the coming decades. However, there are fundamental uncertainties about how and by whom the system will be owned and operated, how its architecture may evolve, how the market and regulatory environments may change, how rapidly and broadly new technology options will be developed and taken up across the system, and how users will impact and be impacted by these changes.
Given the many forecasts and outlooks published by government agencies, national laboratories, scholars, think tanks, interest groups, and countless others, it can be easy to forget how difficult or impossible it is to accurately predict the future of something as complex and interdependent as energy systems. Retrospective analysis of past efforts to make assessments about the future of those systems have demonstrated that even the best projection efforts have frequently turned out to be incorrect. Figure 1.2 provides four examples that document this point. It is easy to produce many more.1
___________________
1 The estimates from the Energy Information Administration (EIA) included in Figure 1.2 are not forecasts. As noted in the Annual Energy Outlook (EIA, 2020), EIA projections out to year 2050 are not “predictions of what will happen, but rather, they are modeled projections of what may happen given certain assumptions and methodologies.” The EIA Reference case assumes that current laws and regulations impacting the sector, including laws that have end dates, will remain unchanged in the future and that it reflects the current views of leading economic forecasters and demographers on issues such as energy production, delivery, and consumption technologies (EIA, 2020). However, while EIA makes it clear that the projections within the reference case are not a forecast, they typically are used with the assessment community as a de facto one. Thus, the difficulties in making accurate projections are critical to note. There are other standard scenarios, including the National Renewable Energy Laboratory (NREL) standard scenarios that are used in forward-looking electricity analysis by NREL and others.
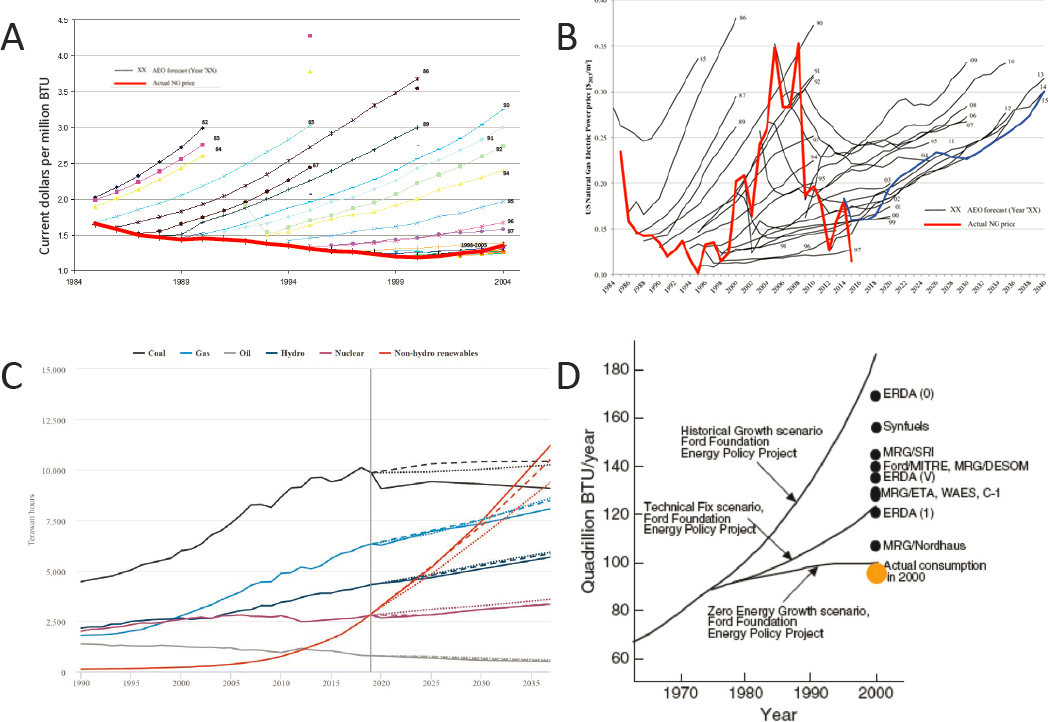
Forecasting the future is complicated by the many possible interactions that can occur among the different parts of complex social and technical systems. Forecasting is made further difficult by the large roles played by shifting consumer preferences, by changes in public policy (Kingdon, 1984), and by major technological, geopolitical, and other events (Baumgartner and Jones, 1993; EIA, 2018).
For example, energy modelers have discovered that it is particularly difficult to predict the prices for the major fuels—in particular, coal and natural gas—that are relevant for the U.S. power sector. As shown in Figures 1.2A and 1.2B, future prices have been consistently overstated by models over the years. The decline in the price of natural gas that has occurred since the revolutions of fracking and horizontal drilling first produced commercially relevant supplies in the mid-2000s has had a transformative impact on electric power supply. Most long-term forecasts leading up to that point largely missed this development.
Similarly, the costs of emergent renewable-energy technologies, such as solar photovoltaic (PV) units, have declined far more rapidly than the cost projections in many forecasts. (See Figure 1.2C.) Demand for electricity has also been difficult to predict beyond the very near term. Profound increases in energy efficiency, for example, have led the economy to use far less primary energy since the mid-1970s than most mainstream forecasts expected at that time. (See Figure 1.2D.)
A recent report from the World Economic Forum (WEF) provides a cogent explanation of why, in the specific case of energy, accurate forecasting is difficult in the specific case of energy systems and markets (World Economic Forum, 2018). This is especially true today, when the energy system is undergoing profound changes (often termed the “energy transition”) resulting from ongoing policy, technological, and behavioral trends. The committee summarizes the WEF’s argument as follows:
- Large parts of the energy value chain are becoming decentralized, flat, and open. This shifts authority and influence away from incumbent firms and infrastructures toward new entrants and even consumers. Many of those new actors, however, do not yet exist, making their impacts on the system difficult to anticipate.
- The most profound effects of large-scale innovation come from interactions of systems. By themselves, each system is hard to forecast, while together the interactions among and within these systems of systems run the full spectrum of unknown impacts and complexities. This partly explains why the track record of mainstream energy forecasting on penetration of new technologies, such as solar, is so poor.
- The energy industry has tended to focus on the inertia of a large system—in terms of size and capital intensity—as a source of stability and therefore predictability. However, that system depends on continued investment. Pervasive uncertainty, including rapidly rising (but still small) market shares for disruptive new technologies, has amplified the risk of deploying capital within incumbent modes. Capital could move quickly even if the legacy physical infrastructure does not. The result of chronic underinvestment may be “creakier” infrastructures and more crisis-driven policy making—amplifying risks even further.
- Many sources of new ideas and technologies lie outside the energy industry, particularly in the realm of monitoring, efficiency, and communications, data analytics and Internet of Things (IoT) technologies. Their impact, for the most part, lies outside the familiarity and forecasting skill technologies. Their foundations and impacts, in many cases, are not familiar to and are beyond the skills of forecasters in the energy industry. The new ideas and technologies come in response to fundamental advances in knowledge along with market needs in other industries. The profound impact of these technologies on energy systems may not arise in response to identified needs within the industry or even the normal forces of supply and demand.
These many sources of uncertainty were highlighted and discussed in a workshop the committee hosted on modeling of the electric system on February 4, 2020. (See the Workshop Summary [NASEM, 2020] and Appendix F in this report.) The committee invited and heard from modelers and practitioners involved directly or indirectly in estimating the future performance of the national and regional generation and transmission systems and local distribution systems. The presentations reviewed the purposes of and technical differences across different types of models, data requirements, and sensitivities to key assumptions. Additionally, participants also highlighted how they and others use the insights from (and limitations of) modeling results as they plan for and make decisions about future investments in their systems.
Even as some players in the electric industry have no choice but to rely on models in order to carry out their responsibilities, a common thread through the presentations was to highlight the limitations of models in the face of high uncertainty about the future, the need to tailor models to the questions being asked, and to communicate the relevance and limitations of modeling results to different types of decision making.
In light of these many considerations, the committee decided not to perform modeling or to use the detailed results of such models in its work. Instead, the committee has chosen to make only a few specific forecasts about how the grid may evolve over the next several decades. The strategy has been to identify a wide variety of factors that could give rise to change, explore and discuss their potential consequences, and then suggest strategies that could be adopted today in order to be better prepared to utilize the range of possible future developments that could unfold.
EVOLVING ELECTRIC INDUSTRY STRUCTURE:
OWNERSHIP, CONTROL, REGULATION, AND INNOVATION
The evolution of the power system from Edison’s Pearl Street Station and a few other isolated systems—the first commercial electric power plants and distribution systems in the United States began operations toward the end of the 1800s—to a vast network that spans the continent, is a fascinating story. It has been described by many others, so it is not reproduced in detail here.2 In many pockets of the global industrial economy, power generation and distribution also took off around the turn of the 19th to the 20th century.3 Today, the U.S. industry includes a large number of investor-owned utilities, four federal Power Marketing Administrations (Bonneville Power Administration, Western Area Power Administration, Southeastern Power Administration, Southwestern Power Administration), several large generation and transmission systems operated by federal agencies, and a large number of systems operated as cooperatives or municipally owned utilities or special purpose districts (e.g., the Salt River Project). The history of the electric industry is full of fits and starts, mergers and consolidations, and technical and regulatory experiments gone right and wrong, and has reflected one of the most massive deployments of capital in the country’s history.
Over a century ago, states began to regulate privately owned utilities, in recognition of the fact that a single supplier of electricity generation and delivery operating over a single geographical territory would be the most economically efficient manner to provide service to consumers (Kahn, 1988). Consistent with this monopoly industrial structure, regulators were charged with ensuring that the rates charged to consumers were based on cost, with capital and other investments deployed in a manner consistent with the public interest. As elaborated in Chapter 3, investor-owned utilities serve roughly two-thirds of U.S. electricity customers, but a much smaller percentage of the nation’s physical area. Much of rural America, and a number of cities, are served by cooperatives and municipally owned utilities, which, because they are governed by boards of directors typically elected by their customers, do not always fall under the purview of state public utility commissions (PUCs) (EIA, 2019). In 2018, even though there were fewer than 300 investor-owned utilities (compared to approximately 3,000 publicly owned utilities), approximately three-quarters of all retail power went through the local distribution systems of investor-owned utilities (EIA, 2019).
Beginning in the last two decades of the 20th century, technological, economic, and policy changes in power generation technology and industrial organization made it possible for new suppliers to provide electricity more economically than some existing power plants. Buoyed by these possibilities, pressure began to build to allow competition in the bulk generation and transmission segments of the electricity supply chain.
The United States, as well as many other parts of the world, responded to these developments in a variety of ways.4 In some geographical locations, and some segments of the industry, policy barely changed; in others, an industry with vertically integrated companies that were long thought to be natural monopolies were transformed into something quite different. States that wanted to allow greater competition took steps to unbundle generation and transmission from retail sales and distribution service for investor-owned utilities in their states. Then, because the U.S. federal government has jurisdiction at the level of transmission and over “sales in interstate commerce,” and in turn pursued policies to support wholesale competition and nondiscriminatory access to transmission, the
___________________
2 See for example, J.A. Cohn, The Grid: Biography of an American Technology (MIT Press, 2018); J.D. Lambert, The Power Brokers: The Struggle to Shape and Control the Electric Power Industry (MIT Press, 2015); J. Jonnes, Empires of Light: Edison, Tesla, Westinghouse, and the Race to Electrify the World (Random House, 2004); J. Riggs, High Tension: FDR’s Battle to Power America (Diversion Press, 2020).
3 For example, G. Hecht, The Radiance of France (MIT Press, 2000).
4 While this report focuses on the United States, it is crucial to note that the same technological, political, ideological, and market forces that led to elements of restructuring in the United States also led to halting, uneven, and highly diverse policy reforms in many other regions of the world. Those experiences in other countries matter to the United States not only because U.S. reforms and reformers helped to inspire some of them (as did restructuring experiments in the United Kingdom, Chile, and a few other places) but also because the structures of overseas markets have an increasing impact on the United States owing to the fact that equipment suppliers are increasingly global firms and thus the products they offer reflect global market conditions, especially in regions that are still rapidly expanding their power systems. On the experience with restructuring across the developing world, see, for example, The Political Economy of Power Sector Reform: The Experiences of Five Major Developing Countries, ed. D.G. Victor and T.C. Heller (Cambridge University Press, 2007), and V. Foster, S. Witte, S.G. Banerjee, and A. Moreno, “Charting the Diffusion of Power Sector Reforms Across the Developing World,” World Bank Group Policy Research Working Paper 8235, November 2017.
industry changed significantly as a combined result of federal and state actions in many parts of the country. Even in states that did not restructure their electric utilities, and with states having jurisdiction over intrastate transmission and pipeline projects, as well as retail- and distribution-level sales and activities, the federal influence on transmission access has been strong. In theory, as technology has evolved, the distribution side of the system, which largely falls under the jurisdiction of states, is also becoming amenable to some forms of competition and restructuring. These options are discussed in Chapter 3.
Competition in power production and supply has been assisted through various policies in recent decades, such as the following (and further elaborated in Chapter 3):
- Federal requirements that utilities purchase power from certain non-utility power production facilities at the utility’s avoided costs (i.e., the cost at which the utility would otherwise produce power);
- Federal support for opening up access to transmission service for other suppliers besides the owner of the transmission lines;
- Requirements in some states that their utilities use competitive supply procurements to identify the most cost-effective new source of power;
- State actions to allow customers to choose their own source of supply, with such supplies delivered through local wires still owned by utilities;
- Federal and many states’ actions to encourage utilities to establish independent system operators and to rely on wholesale power sold at market-based prices;
- States’ and federal policies to allow demand-side resources to compete with generators to ensure least-cost provision of electricity service; and
- State policies to create incentives and/or requirements for power to be supplied by clean-energy resources, including energy efficiency, distributed energy resources located on customers’ premises, and larger-scale clean energy resources (including solar plants, wind resources, storage facilities, and other renewable energy facilities).
These and many other technological, economic, legal, regulatory, and customer-driven changes that are now under way in the electric industry have introduced important and complicated challenges for existing markets and regulations. Some of these changes have even blurred the lines between the activities that are subject to state versus federal regulation, and between the activities where a regulation or a market framework should prevail. The result of all these changes that have occurred at different speeds (or not at all) in different segments of the industry and different parts of the country is that, today, the U.S. power system consists of a mix of restructured and vertically integrated systems, as described further in Chapter 3.
Tensions resulting from these many changes exist not only in the parts of the system where states restructured their electric industries roughly two decades ago to allow for greater reliance on market-based processes. Increasingly, such tensions are also showing up in the parts of the United States that have retained vertically integrated investor-owned utilities regulated by state public utility commissions and the boards of publicly owned utilities, and do not have an independent regional grid operator. A good example is the electric industry in the Southeast, which is experiencing the push and pull from open access to the transmission system (regulated by the federal government) and from large and small customers who either want to choose their power supplier or produce power on their own premises.
In the future, public officials, utilities, and myriad other stakeholders in countless forums will wrestle with decisions on industry structure, on the activities where regulation is necessary, on the conditions that can allow competition to flourish while still allowing for a healthy and reliable power system, and on situations where specific proposals to site electric infrastructure are in the public interest.
Finding 1.1: As spelled out in greater detail in Chapter 3, today the U.S. electricity system consists of a mix of restructured and vertically integrated systems operating in a mix of regulated and competitive market environments. Some systems are investor owned yet regulated in countless ways by public policy; some are publicly owned and regulated more directly through ownership and control (as well as by many state and
federal laws). This highly diverse environment makes it very difficult to generalize about many aspects of the system across the country, engage in coherent system-wide long-range planning, develop a common set of recommendations, or provide advice that is relevant to all parties.
The myriad changes under way in the industry, combined with the committee’s charge, have led the committee to look at what roles innovation will play in the future. As with the industry itself, which is in the midst of profound change along with fragmentation and diversity in stakeholders and regulation, so too the incentives for innovation are in flux. Chapter 4 traces how the incentives that operate at the level of each type of actor in the broader electricity industry affect, as a whole, those patterns of innovation.
For some decades, there has been a “standard” view about the sources and impacts of such innovation. That standard view has emphasized how the dominant position of incumbents and networks, along with risk aversion and heavy regulation, explain why overall technological change in electricity (and energy more generally) is much slower than in other industries such as software and biotechnology. Moreover, the geographical isolation of power systems and the monopoly-oriented structure of the industry has explained why much of the research on innovation has used national markets as the unit of analysis. This standard view, always a simplification, helped to identify expected patterns in technological evolution along with important areas for policy intervention. For example, it suggested that the industry, overall, would not be particularly innovative because innovation could potentially undercut the power of incumbency, and because incumbents faced few if any sources of competition. It suggested that most innovation would come from equipment vendors (who compete for customers, such as utility purchasers of gear, and thus have a stronger incentive for innovation). It also suggested that the industry would be little affected by other industries because most of the products and services in electric power were largely unique to the industry.
Chapter 4 explores how the changing structure of the industry and new sources of innovation have combined to alter many of the incentives and behavior of major actors in the electricity sector. Decentralization and opening of the grid and other networks have created technology and market openings for new types of firms while also creating many new risks. Also important are new sources of innovation that are exogenous to the traditional industry—for example, the Internet of Things, advanced battery storage, communications technologies, and new algorithms for decentralized control of complex systems—that are arising essentially completely outside the power sector and that yet could have profound effects on the grid system. Each of these shifts, by itself, could have profound impacts on the system, and the interactions between the systems could be seismic, raising the level of uncertainty for investment. The last time such policy uncertainties and business-model risks occurred in full force in the electric industry—during the opening up of retail choice in many places across the country during the mid-1990s and early 2000s—utilities’ investments in many parts of their business stalled until cost-recovery considerations were addressed. This being said, there have been some positive early indications from investors regarding utilities shifting generation sources, though the ultimate outcomes remain to be seen.
In particular, Chapter 4 documents how the markets for major power system technologies—such as major power plant equipment, transformers, and control systems—have become global. That has made those markets more competitive, but it has also shifted the interest of major vendors to the most rapidly growing markets, notably in Asia. That may affect design of equipment, including the inclusion of protocols and gear that U.S. purchasers may fear compromise security, and it has affected the geographical location of production. U.S. and European production of heavy electric equipment has atrophied. This globalization in supply chains has expanded the market over which innovations can be appropriated—which may increase the intensity of innovation and possibly competition—but it has also exposed purchasers to supply chain risks expected from “de-globalization” in the wake of trade and investment conflicts, notably between the United States and China.
Finding 1.2: The patterns of innovation and the hardware, software, and system supply chains that have characterized the domestic electricity system in the past are undergoing dramatic transformations that pose a variety of new challenges for industry decision makers, regulators, and national policy makers. Among these changes are the globalization of supply chains. A more global approach offers the opportunity for cooperation that could yield a fuller global investment in innovation. However, it has also exposed purchasers to supply chain and security risks, such as might be expected from “de-globalization” in the wake of trade and investment conflicts, notably between the United States and China.
HISTORICAL VARIATIONS IN U.S. GRID STRUCTURE
Even with similar attributes in architecture, the grid varies in substantial ways across the country, in light of multiple influences. The design of the grid results from many factors such as the size of the customer base, demography and topography of the country or region, availability of fuel resources, and relations between neighboring countries or jurisdictions. Even in the United States, the three interconnections that provide integrated grids in the eastern and western parts of the country, and in Texas, display different designs because of differing population densities, distances between central-station generation and demand, availability of water, coal, natural gas, and renewable resources, and many other factors.
The historical evolution of the electric grid, both from a physical and an economic point of view, influences the current architecture and design of the grid. As noted above, electrical grids started as local distribution networks connecting consumers with local generation plants. Although transmission lines were built quite early to transmit cheaper sources of generation (e.g., hydroelectric power) to distant load centers, the current transmission networks (shown in Figure 1.3) in North America reflect the cumulative additions of transmission to connect and integrate local distribution networks with large central generators. This began in the first half of the 20th century, and continued in the decades since then.
In the 2009 report Americas Energy Future, a previous committee of the National Academies observed that “Most U.S. transmission lines and substations were constructed more than 40 years ago and are based on 1950s’ technology” (NRC, 2009, p. 569). Even in 2020, after 10 more years of aging that infrastructure, that statement remains largely true. Most of these lines were built with the objective of moving power from large central-station fossil, nuclear, and hydro plants, often built in remote locations, to serve load in distant population centers.
Although the physical character of transmission facilities has not changed dramatically in the United States over the past half century, the character of transmission service has evolved significantly in recent years. Over the course of several decades, federal policy has led utilities that own transmission facilities to make the capacity on those systems available to third parties on a nondiscriminatory basis. This was a critical enabler of competition in wholesale power markets.
A 2017 National Academies report explains that:
Today, there is a patchwork of restructured and vertically integrated utilities across the United States. In much of the country, there are hundreds of non-utility entities involved in the power generation, system operations, power marketing, and power trading, and other affiliated activities. The market participants in the electric regions serving two-thirds of the population in the United States are members of organized wholesale electricity markets where a regional transmission organization (RTO) (sometimes called independent system operators [ISOs]) operates the transmission system, prepares regional transmission plans for the market footprint, and conducts competitive product markets (covering energy, capacity, and/or ancillary services markets). (NASEM, 2017, p. 21)
Federal policy and technological improvements have also led to a similar transformation in generation. The bulk power grid supports the delivery of power generated by thousands of power plants located around the country. In 2017, roughly 62 percent of every dollar spent on electricity was tied to its production (with the rest covering costs to deliver the power).5 Figure 1.4 displays how the energy resources used to generate electricity have changed over the past three decades. The biggest shifts in sources of power supply have occurred since the mid-2000s, in tandem with the shale gas revolution and the entry of wind and solar facilities. In 2019, about 4.12 trillion kWh of electricity were generated at utility-scale generation facilities in the United States. Generation fueled by natural gas (38 percent) has now exceeded generation fueled by coal (23 percent) (EIA, 2020a). The drop in generation by coal is partly the result of environmental constraints and pressure to move to a lower carbon generation mix—including the rise of wind and solar power plants that have benefited from incentives at the federal level and other incentives in most states. But the dominant explanation for this shift is rooted in abundant supply of inexpensive natural gas and the associated increase in natural gas-fired electricity generation capacity.
___________________
5 EIA reports that in 2019 the average price of delivered electricity to residential customers was 13.04 cents/kWh, of which generation contributed 58 percent of the cost (7.56 cents/kWh), and transmission accounted for 13 percent (1.70 cents/kWh).
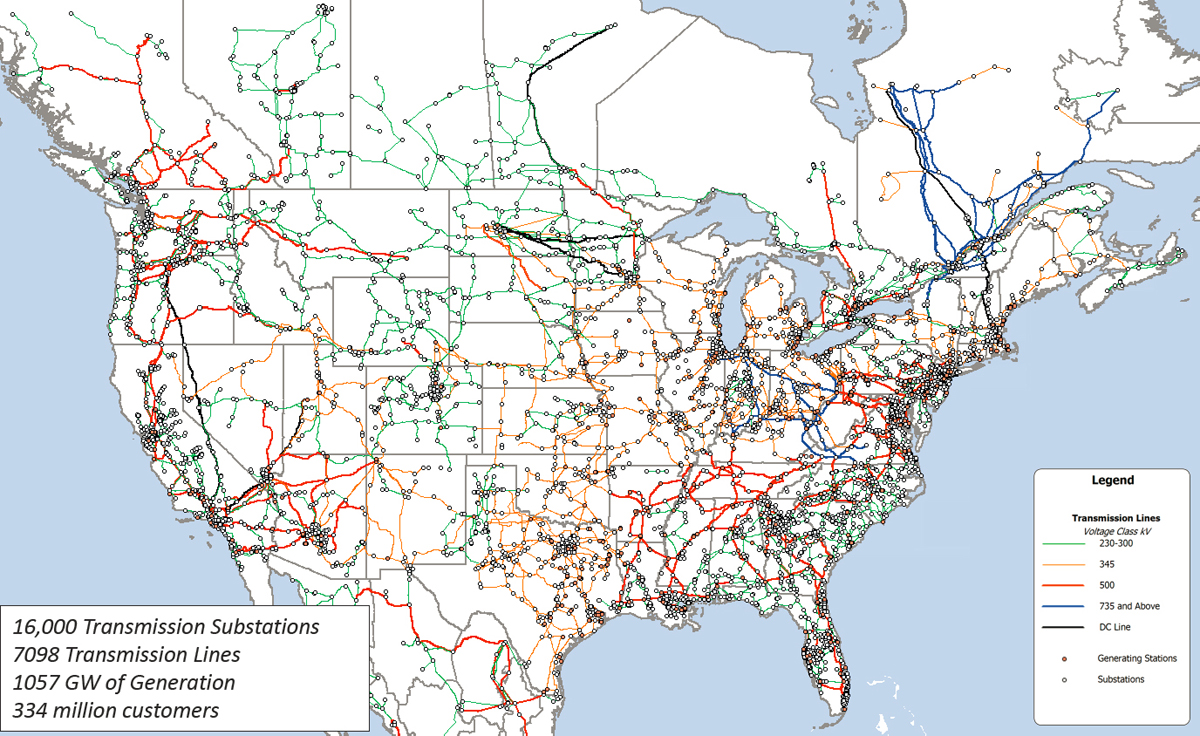
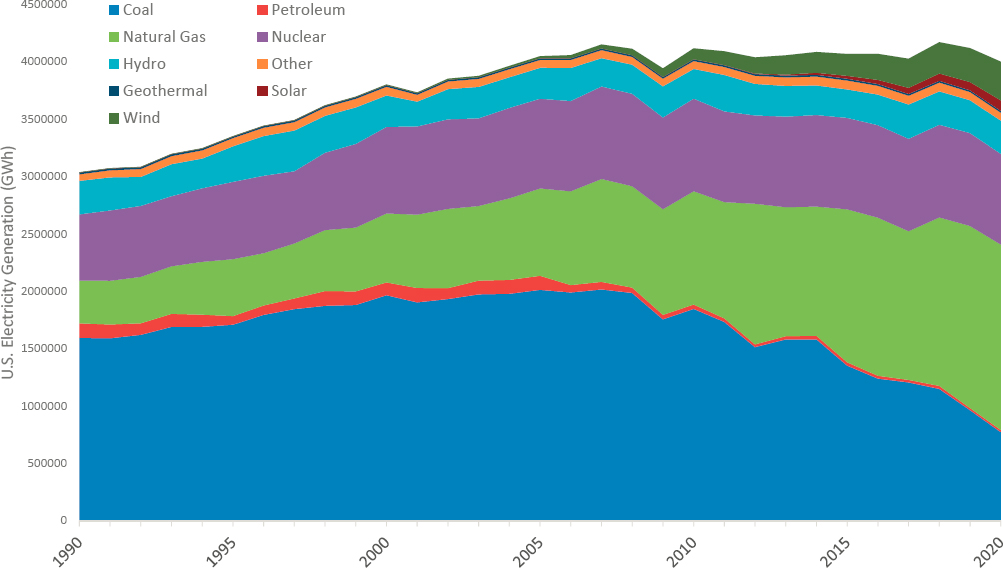
In 2019, just under 20 percent of U.S. bulk electricity supply came from light-water nuclear reactors. The net output of the fleet of nuclear units has remained relatively stable since the mid-2000s, with upgrades of capacity at existing units over that period and recent retirements of several nuclear units in the past few years. However, the fleet is aging. The EIA’s 2020 AEO estimates a decrease in total U.S. nuclear power generating capacity from 98 GW in 2019 to 79 GW in 2050 (EIA, 2020c).
Although wind and solar generation grew by 44 times and almost 200 times, respectively, over what it was in 2001, those two technologies still make up a relatively small share of total electricity generation. In 2019, wind accounted for 7 percent and utility-scale and rooftop solar provided 3 percent of total electricity supply (EIA, 2020c). Together with hydroelectric power (which supplied 7 percent of electricity), these three renewable resources provided approximately 17 percent of total power supply.
All told, total electricity generation from all fossil fuels—natural gas, coal, and oil—has increased by only 2 percent since 2001, while total electricity production from all resources increased by 10 percent from 2001 through 2019 (EIA, 2020a). Fossil generation as a percentage of total power production dropped from 72 percent in 2001 to 63 percent in 2019 (EIA, 2020a).
Finding 1.3: The proportion of electricity produced through burning fossil fuels has declined over the past two decades, but still constitutes the bulk of generation. Gas-fired generation has increased as coal-fired generation has decreased. The amounts produced by nuclear and hydro have remained relatively constant. The share of power from wind and solar has increased steadily in recent years but still accounts for only about 10 percent of total power production.
THE CURRENT STATE OF ELECTRICITY CONSUMPTION IN THE UNITED STATES
Affordable and reliable electricity is a cornerstone of modern industrialized societies. Electricity provides lighting and cooling, powers our digital world, and supports essential services like drinking water and wastewater treatment, public safety, food supply, communications, financial services, and healthcare. Access to electricity is essential to the well-being of households, businesses, and every part of the economy, a fact made abundantly clear after recent hurricanes and heat waves, and during the 2020–2021 coronavirus pandemic. When power goes out even briefly, our daily lives and economy are severely disrupted (NASEM, 2017).
The United States consumes more electricity than any other country except China.6 Even so, on a per-capita basis, the United States still uses three times more electricity than China and most other countries. As shown in Figure 1.5, since the 1960s, U.S. per-capita electricity consumption grew steadily until the early 2000s, after which it leveled off, and per-capita energy consumption started to decline.
Unless the coronavirus pandemic results in unexpected long-term, structural changes in electricity production and use, conventional “business-as-usual” projections forecast U.S. per-capita electricity consumption to decline through 2050 owing to improvements in efficiency (EIA, 2020a). But these futures are quite uncertain. Total demand for electricity could grow dramatically in the future, as a result of, for example, the widespread electrification of transportation and other applications (e.g., in buildings and industry) (Bruckner, IPCC 2014; Getting to Neutral, LLNL, 2020).
Access to electricity has contributed enormously to quality of life and economic productivity in the United States and all global economies. Widespread access to electricity drove economic development in the United States, notably between 1920 and 1950 (Kendrick, 1961). For decades, economic growth correlated strongly with
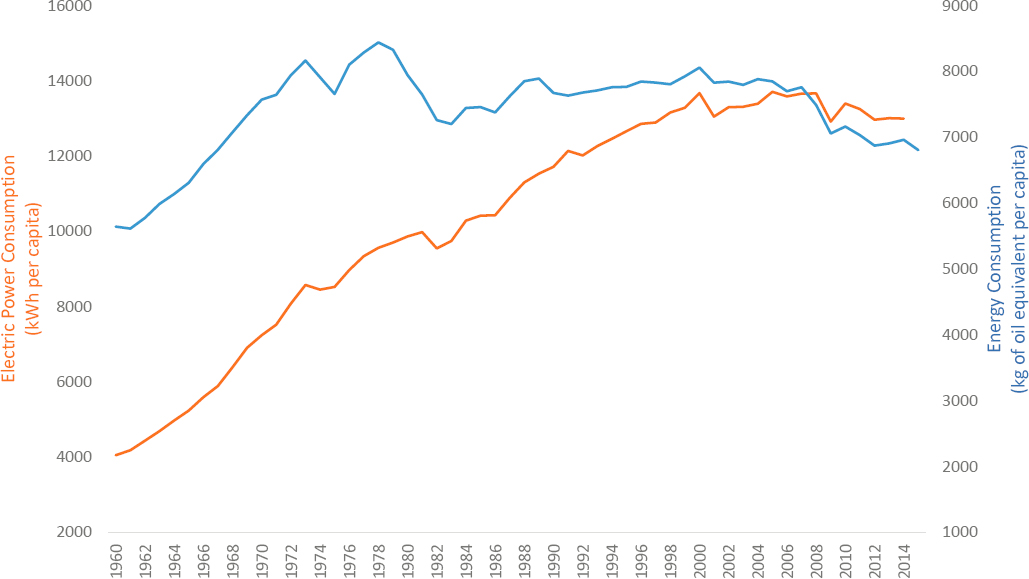
___________________
6 U.S. generation in 2018 was 4,429 TWh, compared to total generation of 7,092 TWh in China (Enerdata, 2019).
growth in electricity use, but that relationship has not held true since the mid-1990s: electricity use has remained nearly flat over the past few decades even as U.S. gross domestic product has risen (Hirsh and Koomey, 2015).
Owing to the development of large-scale, centrally controlled, multistate, interconnected grids, power generation could be spatially separate from loads, supporting both urban and rural economic and industrial development. High-density living and working far from power plants became more feasible, even in hot climates, thanks to assembly lines, elevators, and air conditioning (Victor et al., 2018). The creation of the Rural Electrification Administration (REA) in 1935 allowed electricity to transform life in rural America (Brown, 1980) by facilitating access to air conditioning (Arsenault, 1984), supporting economic growth, population growth, and human health.
By 1940, most houses were “networked” and had become reliant on connections to many services: electricity, gas, telephone, water, and sewer (Gordon, 2016). Today, people are not only networked physically through their connections to utility systems but also, thanks to a myriad of devices, tools, and applications that connect to the Internet, most (but certainly not all) Americans are now networked virtually. The nation’s experience during the 2020 pandemic has made clear how many social and economic activities depend upon electricity, Internet connections, and other supply systems.
With growing numbers of homes and businesses showing interest in generating their own electricity, the “networking” described by Gordon continues to evolve: changing electricity systems are creating innovative connectivity models. Rather than just delivering power from power plants to consumers, the distribution system has the potential to become a two-way system as a consequence of decentralized generation (Figure 1.6). As elaborated in Chapter 2, while these developments could lower costs and improve resilience for some higher income customers, they also have important implications for social equity.
A two-way system may offer benefits related to cost and reliability and “energy independence,” but it also raises safety concerns related to system controls over energized wires, particularly during periods of disaster response. Widespread deployment of distributed energy resources (and resulting two-way energy systems) has not yet materialized but is becoming more technologically feasible.
Further discussion on the drivers of change in the electricity system is presented in Chapter 2.
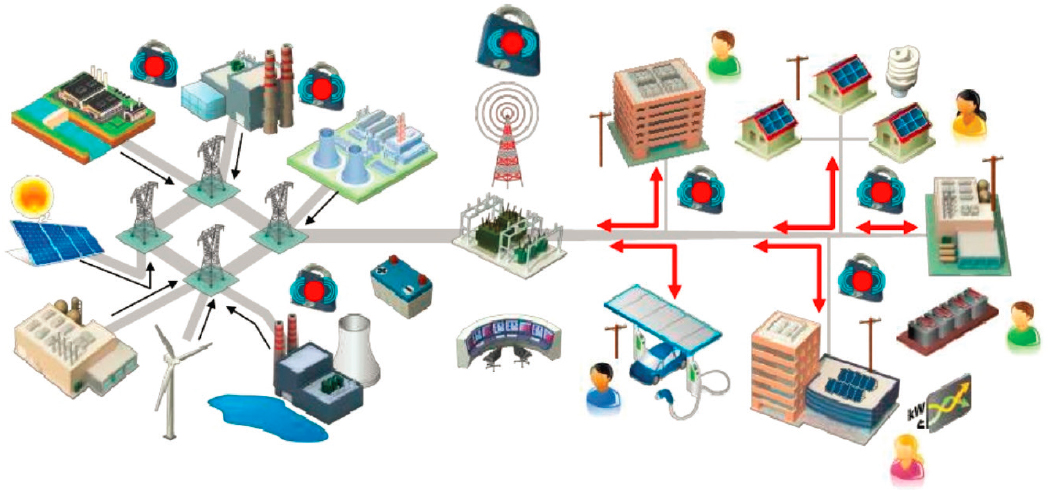
PRESENT U.S. GRID ARCHITECTURE
General Elements of U.S. Grid Architecture
The statement of task for the committee’s work instructed the committee to gather evidence, deliberate, and provide findings and recommendations regarding “both technical and jurisdictional challenges to implementing a broadly applicable approach to grid architectures.”
There is a long history of using concepts related to the architecture of systems to understand how individual components interact and influence each other. Taft (2020) defined grid architecture as the application of system architecture, network theory, and control theory to provide a high-level description of the complete grid and to help understand complex interactions that exist. The grid in its actual operation is an overwhelmingly complex system with millions of individual components and interactions across time domains that span milliseconds to decades and spatial domains from individual customers to synchronization across millions of square miles. Grid architecture spells out in a conceptual sense the connectivity between the different components of the grid, how the different components interact, and rules to keep track of these interactions.
The architecture of the U.S. electricity grid has developed organically over more than a century to meet the nation’s growing needs for electricity. Its structure has evolved from early islanded systems into its current architecture consisting of several interconnected physical and social systems, often called “layers” in the language of grid architecture (Figure 1.7). As shown, these layers encompass the physical components of power systems; the information, controls, and communications components necessary for operations; and the organization components that enable the system to be designed, be funded, and provide services to consumers. The committee’s motivation in depicting the architectural structure of the grid, as displayed in Figure 1.7, is to emphasize that the performance of the grid depends upon (1) the physics of moving electricity from generator to customer; (2) the communications and intelligence for tracking and guiding those flows; and (3) the overall oversight and support to make sure all of this happens in an organized and consistent manner. While Figure 1.7 provides a representation of the overall architecture of the grid, the subsequent section discusses how systems concepts are used in more detailed studies of grid architecture. Chapter 7 expands on the discussion of grid architecture to include a detailed description of these layers and a taxonomy of possible alternative architectures, some more plausible than others.
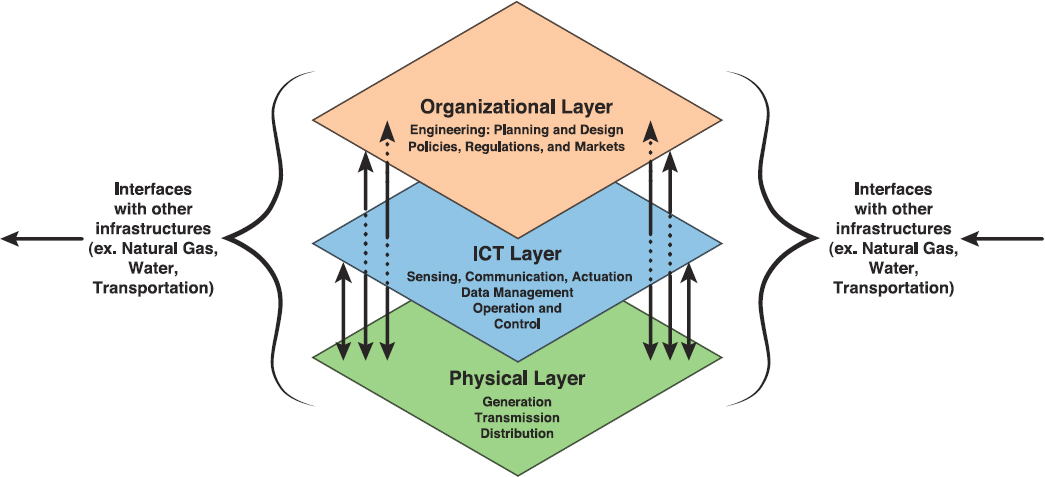
Using the Concept of Grid Architecture to Understand the Electricity System
Grid architecture concepts provide insights into issues associated with grid transformation, including assessing systematic vulnerabilities; identifying and removing legacy constraints and other barriers; managing complexity; assisting in communication among stakeholders; and helping to define new operational platforms (Taft, 2020). In remarks during the public webinar the committee hosted, Taft described the operational alignment of utility and non-utility assets as one transformation issue being addressed using grid architecture. While some forms of non-utility generation have been around for a long time (commercial and industrial on-site electricity generation and independent power producers), the need to increase the focus on grid coordination is heightened by the rise in deployment of distributed energy resources (DER), an increase in controllable loads, and ubiquitous connectivity, as well as the fact that distributed generation and responsive demand assets may not be owned or directly controlled by the utility. By calling attention to the need for greater information and coordination, the committee is not passing judgment on the desirability of these changes. Rather, the point is that such transformative changes need to be accompanied by forms of information sharing as part of the architecture of the grid.
The traditional architecture of the grid is a centralized approach in which the transmission system operator (TSO) performs all system coordination and control while the distribution system is relatively passive, carrying the power from transmission substations to loads. Although this centralized architecture is accommodating some coordination and control by the TSO of some DER on some distribution systems, it is generally believed that such an architecture will not be adequate to ensure reliability when a large fraction of energy resources are on distribution feeders. In that case, it will be preferable, if not necessary, for a layered approach in which the distribution system operator (DSO) has a significant role in coordination with the TSO.
At the committee’s workshop on this topic, De Martini (2020) described how grid coordination using several proposed system designs could play out in different electric systems. The industry will need to find new architectures for such TSO-DSO coordination to minimize operation problems, such as through the most efficient routing of information flows or control paths that are needed to meet the performance requirements for such coordination and control by both the DSO and TSO.
Grid Architectures: Overview and Trends
Of the three layers in Figure 1.7, the physical layer is made up of all the generation, transmission, and distribution assets that move energy from the generation source to consumers. The physical layer is overlaid with the ICT layer that monitors and controls the power grid; the assets consist of ICT, data management, and operations software. Both the physical and ICT layers have oversight through an organizational layer consisting of engineering principles and standards for planning and design, and policies like organizational goals for increasing decarbonized resources and regulatory oversight of markets and tariffs. The electric infrastructure is also connected to other infrastructures like gas, water, railways, and various industries, so the electric grid architecture is influenced by these other complex systems.
The following presents some of the trends in all the layers of the grid architecture that are being precipitated by advances in technologies. As each layer is quite complex, being made up of many components, only selected features are discussed in terms of the trends that are observed today. These trends are not specific enough to do proper forecasting of the grid architecture with any certainty, but they do provide some indications to develop possible future scenarios of what the architecture may look like.
Physical Layer of the Grid Architecture
Generation
While central generation is still the dominant source of electricity for most of the power system, the fraction of DER in the system is growing. Unlike central generation, which has always been connected to the high-voltage network, DER are typically connected to the distribution network, sometimes on the customer side of the meter.
Morever, unlike many central station generators that use rotating machines that contribute stabilizing inertia to the system, many of the newer energy resources—both DER and some new central-station generators like wind and solar, are connected to the system using power electronics and at present do not contribute inertia. Thus, the proportion of rotating mechanical inertia is decreasing in some parts of the system, affecting the synchronizing stability of the electrical grid. As this happens, frequency and voltage controls have to be provided by the power electronic interfaces.
The adoption of both DER and utility-scale wind and solar to decrease emissions of conventional air pollutants and CO2 will likely intensify. This change is having repercussions in all of the physical and other layers of the architecture. The adoption of both DER and utility-scale wind and solar to decrease CO2 emissions will likely intensify. This change is having repercussions in all of the physical and other layers of the architecture. This is because the control of the grid performance, which is largely dependent on the central generators, will have to be accomplished increasingly by the DER, thus requiring changes in both the ICT layer and the organizational layer.
Transmission Network
Today, the transmission system is largely a network of three-phase AC transmission lines with voltage levels that range up to 765 kV. There are also a number of DC back-to-back connections between synchronous regions. There are a few long point-to-point high-voltage DC lines, although the United States has taken less advantage of HVDC than other regions of the world. This may change as newer power electronics, discussed in Chapter 5, are adopted.
To the extent that new transmission lines or expanded capacity of existing transmission corridors are being pursued, today this is mainly to increase resilience or to mitigate potential reliability violations. It is much harder to add facilities to relieve congestion or to connect remotely located utility-scale renewable facilities to the grid. As electrification is pursued for decarbonization, expanded transmission capacity may become increasingly important and other layers (e.g., in the organizational layer, which includes policy and regulation) will need to evolve to accommodate such changes. And, because newer transmission is likely to be smarter, more observable, and controllable, it will need to be supported by technological advances in the ICT architecture.
Distribution Networks
Many distribution systems are undergoing some of the most rapid changes in the grid. Distribution system automation is becoming widespread. Small-scale generation is now being connected to distribution feeders, and more customers, especially those with commercial buildings and industrial loads who want to lower costs, are willing to curtail their load on demand. While there are legal and regulatory constraints, some customers are forming their load and generation into microgrids to provide more resiliency by operating as an island during emergencies.
These developments raise new operational issues for the distribution feeders. If there are enough DER on a feeder, the direction of current will sometimes be toward the substation from the customer (Antonova et al., 2012). Feeder relays, fuses, and safety devices must be reconfigured for such two-way flow. Increased charging of electric vehicles (EVs) can present heavy loads that cluster in certain neighborhoods and require feeder upgrades. The more complex operation of the distribution system calls for increased automation.
As generation is spread over both transmission and distribution, the frequency and voltage control of the whole system is impacted by all of the generation, transmission, and distribution across the physical layer. As this happens, the functions now performed separately by TSOs, DSOs, and distribution-level DER consolidators must be coordinated and ways found to communicate and control the combined system. While this could be done by having the TSO take over the entire function, a more likely outcome is some form of collaborative control between the TSO and DSO to jointly manage distribution-level DER. Such architectural choices will affect the economic and secure operation of the grid and should be carefully designed to suit the many parameters of this physical layer.
Finding 1.4: When generation was mostly central and only connected to the transmission layer, the distribution layer could be designed and analyzed separately from the generation and transmission layers. With
generation increasingly connected to the distribution layer, the frequency and voltage control of the whole system is impacted by all of the generation, transmission, and distribution across the physical layer. Improved simulation tools would be needed to address these changes. Recommendations for developing those tools are provided in Chapter 5.
ICT Layer of the Grid Architecture
Sensing, Communication, Control, and Actuation
As elaborated in Chapters 5 and 6, the transmission system is operated by many control centers, known as Energy Management Systems (EMS), each controlling a portion of the transmission grid. The balancing of generation and load in its own area is done automatically by the EMS. The operator at the control center can monitor real-time measurements from the system and open and close circuitbreakers to change the topology of the transmission network.
The bulk power system is well instrumented, with a strong communication backbone, and is closely managed by RTOs/ISOs and utilities and has high levels of reliability. However, distribution-system reliability levels are more varied and generally lower, with an average of 4 hours of outages every year. As information technology has become more affordable, more measurements, communications, and computers have been added to the distribution grid to improve local reliability. Today, distribution management systems (DMS) are common and are increasing in functionality. The typical scan rates for distribution measurements are slower but allow the DMS to control voltage or sectionalize distribution feeders automatically.
Control rooms at most substations are now full of microprocessors because protective relays and instrumentation are increasingly digital. Thus, data can be stored at substations as well as with the EMS or DMS. The communication system can now be a network connecting all the substations, rather than a star connection to the control center, thus providing redundancy in data paths. The ability to communicate between substations is also enabling wide-area controls and protection that was previously limited to the local substation.
As phasor measurement units (PMUs) are being installed at transmission substations, higher bandwidth communication is needed to handle the scan rates, which are in milliseconds rather than seconds. For the distribution system, more automation will require more measurement sensors along the feeder. Also, where advanced metering is present, its data can be useful for DMS functionality if available in near real time. In general, the information system that overlays the power grid can utilize more of the advances in computers and communications.
The design of the information infrastructure is held back by the absence of standards and simulation tools that would allow more effective use of the growing numbers of sensors and communications and computer networks. As better simulation tools are developed, they need to be made compatible with simulation tools for the physical layer of the system as recommended in Chapter 5.
Data Management
Centralizing operational data at the EMS and DMS was necessary when they housed the only computers on the system. Today, with thousands of microprocessors scattered in every substation and along distribution feeders, data can be stored wherever most needed. The quality of the data has always been an important consideration, but with the large increase in the quantity of data and its exchange, the management of the data is now a concern.
The structure of the database that hold these data is usually proprietary to vendors, and although there is an international standard—the Common Information Model (CIM)—the utility industry in the United States has not insisted that their systems be based on this standard, making data exchange between the EMS and DMS that control different portions of the same grid cumbersome and slow.
Many trends, such as more technology at the grid edge, cannot be sustained without new operation and control applications, which will require better data management. For at least several decades, human operators will still be at the control centers and data for their use will have to be centralized. However, many data streams arrive too quickly or are too voluminous for direct use by central operators, and the processing of these data can be done
closer to the source rather than at the EMS/DMS. A more distributed database based on common standards will have to be developed if more distributed operation is expected or desired.
In addition to the control and operation of the grid, its design and planning require the use of many analytical tools that have to be run on very large databases that describe the grid with all its assets and technologies. The U.S. utility industry lacks a common standard for such databases and instead uses proprietary databases that are designed by different vendors for different analytical tools. The lack of interoperability between the databases of the interconnected power companies makes it particularly inefficient and expensive to make improvements in the planning and operation of the grid that require the use of software tools that do not communicate with each other. As computer science advances the use of artificial intelligence and machine learning, the promise of using these tools for better planning and operations can be achieved only with a modernized data management infrastructure.
The data management layer of the architecture—the software part of the ICT layer—also suffers from a lack of standardization and simulation capability.
Operation and Control
Most applications at EMS/DMS are advisory to human operators and do not run closed-loop. The operator can monitor the system data in real time and the EMS/DMS have applications that perform state estimation, contingency analysis, and voltage optimization that can inform the human operators in their decision making. The only automatic control at these control centers is the control of frequency and voltage.
Faster controls and protection applications are all local and do not require communications. With the availability of higher bandwidth communications, measurements can be scanned much faster, and sent to a controller in a different substation, thus allowing wide-area fast control. Special cases of wide-area control, called special protection schemes (SPS), are already in use especially for preventing instability. These will be increasingly used because upgrading the information architecture with more measurements, communications, and controllers will make such control easier and cheaper to implement.
As the number of generators with their power electronic interfaces and the number of other controllers (for voltage control and line flow control) proliferate, dependence on human operators will have to decrease. Other than the sheer numbers of controllers, the power electronic controls are also much faster, so much of the new controls will have to be automatic.
Finding 1.5: The interconnected grid will likely transition from hundreds of controllers today to millions of controllers in the future, which will greatly increase the interactions between those that are electrically close. These kinds of distributed controls to control an interconnected grid will be new and can be designed in many different ways, as presented in Chapter 5, and some of the resulting control architectures may be transformational. The performance and design of such pivotal control architectures will require more flexible simulation tools that do not exist today.
Organizational Layer of the Grid Architecture
Policies, standards, and regulations can radically affect the architecture of the grid, and as these can vary regionally, the grid also evolves differently across the country. Thus, the organizational decision making is a fundamental layer of the grid architecture.
Engineering: Planning and Design
Before electricity generation was restructured, bulk generation was jointly planned with transmission. Generation can now be built by multiple parties. However, in many regions joint planning of generation and transmission is largely absent.
Transmission-system planning now has to anticipate the possibility of generating units being developed at various locations, with specific transmission project plans often arising in the context of specific interconnection
requests. When asked to consider expanded transmission, many planners instinctively considered new lines. However, options such as reconductoring, circuit addition, increasing operating voltages, or converting HVAC to HVDC using existing rights of way may involve lower costs and face lower regulatory barriers (Reed et al., 2020). Adding a completely new transmission line often takes 10 years or longer. Many of those who build gas, wind, and solar generation have much shorter construction schedules. Hence, broad regional and long-term transmission-system planning now requires forecasting various generation build-out scenarios.
Distribution planning in the past only needed to anticipate local population and economic growth, with the flow of power always going from the utility substation to the customer. With more and more DER located on the customer side, it is more difficult to forecast what the loading profile will be on distribution feeders even 5 years into the future. Storage and EVs complicate this challenge.
Thus, the planning of transmission and distribution has become more complex today, and planning tools have not kept up with these complexities. In addition, with the ability of the generation developers and customers to make independent choices that impact the grid, local distribution utilities are no longer solely responsible for how the grid evolves. As such, no organization appears to be the backstop for guaranteeing grid reliability across both the bulk power and local systems.
Finding 1.6: Because no single entity will design the grid of the future, policy guidelines are needed, as noted in Chapter 3. Those guidelines, supported by appropriate analytical tools as noted in Chapter 5, must help to avoid untenable and unreliable designs, or designs driven by the interests of individual actors in which total costs are much higher than they need to be.
Policies, Regulations, and Markets
Public policy affects the form and functioning of the electric grid, and these policies and regulations are constantly evolving. Obviously, policies on the levels of sustainable and reliable electricity production directly influence activities in the industry, while the policies on forest-fire mitigation and containment and on taxes may also do so.
As discussed in Chapter 3, the utility industry involves a mixture of publicly owned companies governed by their boards and investor-owned utilities (IOUs), with different forms of governance and regulations. The interstate trading and transmission of electric energy and services is regulated by the Federal Energy Regulatory Commission (FERC), as is the reliability of the bulk power system. States have jurisdiction over the retail sale of electricity as well as the reliability of the distribution systems of IOUs by essentially setting standards for and controlling the investments in distribution networks. The proliferation of DER and controllable loads has complicated the reliability, operational, and economic issues of local systems, and the regulatory boundaries of FERC and the states now overlap in many respects. The expansion of DER in combination with regulatory decision making will influence the planning and evolution of the grid’s future architecture.
The FERC regulations have essentially influenced the design of the wholesale markets of electric energy and services. As energy resources—generation, storage, and controllable loads—have increased in the distribution system, the FERC market regulations have been expanded to handle the distributed resources within the wholesale markets. However, the distribution system has been under the jurisdiction of state regulators who could set up local retail markets that would operate in parallel with the wholesale markets (as discussed in Chapter 5).
The electric grid architecture has always interacted with many other sectors of the economy. The transformation of the transportation sector toward more EVs will have a direct impact on the grid. Water treatment and delivery depends on reliable power supply, and output at many thermal generating stations depends upon access to water for cooling purposes. Consumers’ access to commercial communications systems affects their ability to offer price-responsive demand on to the grid. Fuel supply chains obviously affect the power system. With increasing reliance on solar and wind resources, output is much more volatile than, for example, the availability of coal or gas, making the interaction with the electric grid less stable. Similarly, the development of electric storage technologies will directly impact on the operation of the grid.
Finding 1.7: Ongoing architectural changes have interdependent relationships with changes in technology, policy, and other infrastructure systems. Studying these relationship changes and their implications for electric system performance relies on modeling scenarios. For example, the proliferation of distributed generation and demand response requires changes in the auction market bidding, clearing, and settling procedures. Similarly, where other related infrastructures—such as the natural gas pipeline system—become a major constraint on operating the electric grid, policy makers need to consider the trade-offs in making regulatory changes across such infrastructures.
STRUCTURE OF THIS REPORT
Chapter 2 describes the drivers of change in the electric power system, beginning with changes in the nature and mix of future demand, the need to achieve deep decarbonization, edge-of-grid developments including distributed generation, storage and energy management resources, efforts for and challenges from the effort to achieve deep decarbonization, challenges arising from high penetrations of nondispatchable sources of generation, efforts to address social inequity and energy poverty, changes in labor markets within the electricity industry, as well as the market forces experienced by producers and users of electricity from shifts of relative costs of different energy system technologies.
Chapter 3 builds on the discussion of society’s expectations for power systems and examines how legal and regulatory frameworks are changing to accommodate a rapidly changing electricity system. It describes the history of regulatory frameworks across different regions in the United States and points out trends in generation, transmission, and distribution planning, as well as the growing possibility of grid-edge distributed resources combining to form microgrids.
Chapter 4 describes the important role that investment in technology and innovation have in shaping the outcomes of the future grid system. This chapter will cover the economic challenges involved in devoting capital to R&D in the electricity industry, and the industry’s ability to initiate large-scale technical changes.
Chapter 5 describes the technical details of key emerging technologies and capabilities that could shape how the grid develops, including advances in energy efficiency and ways to address challenges related to the intermittency of renewable resources. The future grid must be resilient to disruptions rising from natural events, physical security risks, and (in particular as the grid becomes increasingly complex, interconnected, and incorporates more digital technology and communications systems) cybersecurity.
Chapter 6 discusses physical and cyber risks, assesses the current threat landscape, and outlines approaches to mitigate the threats on grid reliability and resilience.
In each chapter, the committee makes many specific recommendations on how grid modernization can occur while continuing to maintain and strengthen an electric power system that is safe and secure, clean and sustainable, affordable and equitable, and reliable and resilient.
The final chapter (Chapter 7) integrates the findings of the whole report and contains overarching recommendations that highlight what the committee believes to be the most important factors to consider as the nation develops a future grid system that benefits all Americans. These five major needs are to improve our understanding of how the system is evolving; ensure that electricity service remains clean and sustainable, and reliable and resilient; improve understanding of how people use electricity and sustain the “social compact” to keep electricity affordable and equitable in the face of profound technological changes; facilitate innovations in technology, policy, and business models relevant to the power system; and to accelerate innovations in technology in the face of shifting global supply chains and the influx of disruptive technologies.
REFERENCES
Arsenault, R. 1984. The end of the long hot summer: The air conditioner and southern culture. Journal of Southern History 50(4): 597–628.
Baker, S.E., J.K. Stolaroff, G.Peridas, S.H. Pang, H.M. Goldstein, F.R. Lucci, W. Li, et al. 2020. Getting to Neutral: Options for Negative Carbon Emissions in California. Lawrence Livermore National Laboratory. https://www-gs.llnl.gov/content/assets/docs/energy/Getting_to_Neutral.pdf.
Baumgartner, F.B., and B.D. Jones. 1993. Agendas and Instability in American Politics. Chicago: University of Chicago Press.
Brown, D.C. 1980. “Electricity for Rural America: The Fight for REA.” OSTI.Gov.
DeMartini, P. 2020. “Operational Coordination.” National Academies Webinar Presented at the Grid Architectures from Theory to Practice. Washington, DC. January 29.
EIA (U.S. Energy Information Administration). 2018. Annual Energy Outlook (AEO) Retrospective Review: Evaluation of AEO2018 and Previous Reference Case Projections.
EIA. 2019. “Annual Energy Outlook 2019 with Projections to 2050.” January 24, 2019.
EIA. 2020a. Electric Power Monthly. August 2020. Table 7.2a (2019 data), and EIA Data Browser (monthly data, 2001). https://www.eia.gov/totalenergy/data/monthly/archive/00352008.pdf.
EIA. 2020b. “Today in Energy.” https://www.eia.gov/todayinenergy/detail.php?id=42755#:~:text=In%20the%20AEO2020%20Reference%20case%2C%20total%20U.S.%20nuclear%20power%20generating,occur%20between%202020%20and%202025.
Enerdata. 2019. “Electricity Production Data. World Electricity Statistics. Enerdata.” Global Energy Statistical Yearbook. https://yearbook.enerdata.net/electricity/world-electricity-production-statistics.html.
Gordon, R.J. 2016. The Rise and Fall of American Growth. The Princeton Economic History of the Western World.
Hecht, G. 2000. The Radiance of France. Cambridge, MA: MIT Press. https://mitpress.mit.edu/books/radiance-france.
Hirsh, R.F., and J.G. Koomey. 2015. Electricity consumption and economic growth: A new relationship with significant consequences? Electricity Journal 28(9). https://law.stanford.edu/wp-content/uploads/2016/06/Electricity-Consumption-and-Economic-Growth.pdf.
Kahn, A.E. 1988. The Economics of Regulation: Principles and Institutions. Vols. 1 and 2. Cambridge, MA: MIT Press.
Kendrick, J. 1961. Productivity Trends in the United States. National Bureau of Economic Research. Princeton, NJ: Princeton University Press.
Kingdon, J.W. 1984. Agendas, Alternatives, and Public Policies. New York: Little, Brown.
NASEM (National Academies of Sciences, Engineering, and Medicine). 2017. Enhancing the Resilience of the Nation’s Electricity System. Washington, DC: National Academies Press. https://doi.org/10.17226/24836.
NRC (National Research Council). 2009. America’s Energy Future: Technology and Transformation. Washington, DC: National Academies Press. https://doi.org/10.17226/12091.
NRC. 2012. Terrorism and the Electric Power Delivery System. Washington, DC: National Academies Press.
Reed, L., M. Dworkin, P. Vaishnav, and M.G. Morgan. 2020. Expanding transmission capacity: examples of regulatory paths for five alternative strategies. Electricity Journal, 33:106770.
Taft. J. 2020. “Thinking about the Whole Grid: Grid Architecture.” National Academies Webinar, presented at “Grid Architectures from Theory to Practice.” Washington, DC. January 29.
World Economic Forum. 2018. Transformation of the Global Energy System. https://www.weforum.org/whitepapers/transformation-of-the-global-energy-system.