5
Science Enabled by the Space Environment
Some of the most amazing things known about life on Earth and in this universe cannot be learned by experiments conducted solely on Earth. Space-enabled fundamental science represents the opportunity to understand fundamental biological and physical mechanisms that cannot be studied on Earth, and that can only be understood by access to the space environment beyond this planet. From recent history, it is clear that such knowledge helps society not only better understand our place in the universe with a sense of awe but also take major technological leaps forward to make daily life on Earth safer and easier.
As discussed in Chapter 1, the reduction of gravitational forces and high levels of ionizing radiation are two major factors distinguishing the space environment from terrestrial conditions. Gravity causes buoyancy, which profoundly alters flow motion, material transport, and segregation in both biological and physical systems. In the familiar gravity on Earth, particles settle to a plane; microgravity releases them to circulate in a three-dimensional space. In addition to reduced gravity, the radiation and chemical environment of space is unique, and variable, depending on the distance from Earth and the nature of the environment. Other space environment features related to electromagnetic field strength, presence of dust particles, and cycles of temperatures and pressures far beyond the “standard temperature and pressure” that serve as Earth’s baseline for experiments also play a role (see Chapter 1). The systematic difference in transport phenomena as a function of variable gravity enables solid materials and fluids to react differently with each other, invoking different distance and time scales, and affecting kinetics and dynamics. These features unique to the space environment and inaccessible on Earth enable the search for novel synthetic pathways and new materials and chemicals made in space and can also surprise scientists with non-intuitive behavior governing transport phenomena. Furthermore, microgravity in low Earth orbit (LEO) and beyond enables careful studies of situations that are not at thermodynamic equilibrium, and thus not well predicted by existing theories and equations—for example, studies of colloidal systems such as active particles (Khadka et al. 2018) designed to serve as microscopic models for interactions among living objects such as birds, fish, bacteria, and people.
Chapter 5 is thus organized around a single theme that presents the priorities of science for the coming decade:
Probing Phenomena Hidden by Gravity or Terrestrial Limitations: Revealing underlying biological and physical processes that cannot be quantified on Earth.
This theme describes the space-enabled fundamental science that will form the underpinning of both space-enabling and Earth-based applications for future generations. Measuring, modeling, and understanding these phenomena are sometimes referred to as fundamental science; the primary motivation is deeper understanding of how the world and universe around us works. While the related research may seem abstract or academic, such fundamental science is the “seed” for crops of end-use applications and practical benefit to society that historically follow over future decades. This utility may take a human lifetime to manifest, but who can doubt the value of investing in that anticipation (Simmons 2014) with examples of that promise in today’s energy storage materials (including now ubiquitous electric vehicle batteries dreamed up more than 20 years ago), medicines (including proteins that bind with precision based on knowledge of nucleic acid sequences), and navigation technologies (including data from the space-based radio-navigation system connected to every mobile smart phone)? Indeed, this fundamental knowledge—the understanding that will become the stuff of textbooks and cutting-edge products for the next Earth-based generations—also advances space exploration when harnessed in an engineering context. That is, the research that is enabled by access to space eventually—and sometimes quite predictably and quickly—also enables new approaches to travel between, communicate across, and live in space environments.
Finding 5-1: Fundamental research in biological and physical sciences serves the basis of many indispensable societal capabilities and conveniences on Earth, in addition to allowing society to understand more about how the surrounding world works.
The space-enabled scientific questions within this theme are complementary to and often intersect the space-enabling scientific questions described in Chapter 4, which are in turn organized thematically around Adapting to Space on slipping the bonds of Earth, and then Living and Traveling in Space for longer durations and over greater distances from Earth. This chapter focuses on the key scientific questions (KSQs) that are enabled by access to space environments including and beyond LEO, as well as the rationale and possible research approaches for such investigations.
Chapter 5, organized thematically around Probing Phenomena Hidden by Gravity or Terrestrial Limitations, includes KSQs related to biological phenomena that can be understood completely only when some features of the space environment are considered. However, this chapter also places emphasis on KSQs regarding physical phenomena, the answers to which impact human life but may be entirely about abiotic or engineered materials. Table 5-1 summarizes these four KSQs enabled uniquely by access to space environments.
These four KSQs admit some overlap with one another, regarding experimental systems of interest or method of inquiry. This can be viewed as a strength in identifying broadly relevant scientific needs. These questions also inherently cross disciplines, requiring knowledge from physics and chemistry to materials science and engineering. The questions apply to many classes of materials and phenomena, including biophysical phenomena, and are also relevant to the development of space-based technologies of broad utility, from autonomous spacecraft navigation to improved life-support systems for humans in space. Quests for biological science principles need to be aware of and draw from physical principles; and impactful physical science-motivated inquiries need to be aware of and leverage knowledge gained from analogy to biological systems. This is not a novel concept: the electrochemistry of today’s portable batteries was inspired in form and function by the “animal chemistry” stored in twitching
TABLE 5-1 Key Scientific Questions Enabled by Access to Space Over the Decade 2023–2032
Theme | Key Scientific Questions |
---|---|
Probing Phenomena Hidden by Gravity or Terrestrial Limitations: Revealing underlying biological and physical processes that cannot be quantified on Earth |
|
animal muscles (Tretkoff 2006). Living organisms, intrinsically out of equilibrium (as an organism at equilibrium is dead), have harnessed and refined physical and chemical processes for biological function to sustain homeostasis, so there is strong overlap between biological and physical sciences in any discussion of fundamental phenomena, especially involving transport, soft matter, interfaces, heterogeneity, and phase separation. Fundamentally, there is strong overlap between physical and biological phenomena, especially in the area of interfaces, phase separation, self-assembly and colloids; materials synthesis and processing are intertwined with heat and mass transport; and organic and inorganic materials span interatomic interactions ranging from weak to strong, with many properties in common. Each KSQ takes the form of a fundamental question, followed by a discussion of scientific impacts and rationale, as well as potential research approaches in space. Advances in answering these KSQs rely strongly on access to computational tools and databases.
BPS KEY SCIENTIFIC QUESTIONS THEME 3: PROBING PHENOMENA HIDDEN BY GRAVITY OR TERRESTRIAL LIMITATIONS—REVEALING UNDERLYING BIOLOGICAL AND PHYSICAL PROCESSES THAT CANNOT BE QUANTIFIED ON EARTH
This theme can be referred to in shorthand as phenomena hidden by Earth’s environment. The path to identifying the scientific principles otherwise obscured by 1 g gravity, relatively low radiation levels, and other features that define “standard conditions” of Earth-bound research is guided by the following four KSQs:
- What are the mechanisms by which organisms sense and respond to physical properties of surroundings and to applied mechanical forces, including gravitational force? To address this fundamental question thoroughly, including the capacity to cope with or leverage differences in those properties and applied forces in space environments, sustained access to the space environments of interest is required. As just one example, the response to gravitational forces by all organisms ranging from microbes to plants to mammals occurs along a spectrum of gravity (not just 1 g and 1/6 g), so mechanistic answers require access to that spectrum.
- What are the fundamental principles that organize the structure and functionality of materials, including but not limited to soft and active matter? The interatomic and electronic forces that govern the structure and many properties of materials on Earth are well understood at standard temperature and pressure for many material classes including elemental metals. However, in the altered space environment and in the context of complex alloyed, inorganic, and organic materials (so-called soft matter or active matter, whether natural or synthetically engineered), as well as their solid composites or fluid-solid suspensions, these fundamental principles have been elusive. The space environment (including microgravity) allows for control or relaxation of the physical forces that obscure those principles.
- What are the fundamental laws that govern the behavior of systems that are far from equilibrium? Classical thermodynamics predicts the stability of phases based on laws established to describe the energy or work of a system, as well as the reversibility of that system shifting from one equilibrium state to another. When systems or materials are from that equilibrium state, as can occur under high rates of change in pressure or temperature, assumptions of how work and energy are exchanged or how heat is dissipated can fail miserably. To harness such states, new understanding is needed.
- What new physics, including particle physics, general relativity, and quantum mechanics, can be discovered with experiments that can only be carried out in space? The space environment facilitates experiments impossible on Earth, including those required to understand dark matter and dark energy. The current “standard models” of physics and relativity fail to predict and describe these dominant features of the universe. Moreover, the currently incomplete knowledge of atomic, molecular, and optical physics and phenomena such as quantum entanglement also gate progress in technologies needed for space communication and navigation, motivating practical implications of such fundamental understanding beyond the coming decade.
These KSQs are further described below, in terms of the impact and rationale motivating this use of the precious space environment resources for fundamental research. Possible research areas addressing sub-questions and
specific systems of study are also described, with full understanding that these are a subset of the possible research areas that will best address these KSQs in the coming decade of access to space for BPS research.
Recommendation 5-1: NASA should substantially increase resources dedicated to producing and understanding the answers to the key scientific questions detailed in this report. This investment recognizes the potential for significant societal impacts utilizing the space environment for the biological and physical sciences portfolio in the coming decade, aimed at
- Identifying the mechanisms by which organisms sense and respond to the surrounding environment, including gravitational force;
- Advancing knowledge of material structure, self-assembly, and stability of materials, including but not limited to soft/active matter, in space environments, cognizant of but distinct from the applications of that knowledge to space exploration and habitation (e.g., manufacturing in space);
- Supporting ground-based and microgravity research on understanding the fundamental laws of systems far from equilibrium, especially those that underlie the existence of life; and
- Identifying new principles of physics that can only be discovered through experiments in space, including those governing particle physics, general relativity, and quantum mechanics.
Question 8: What Are the Mechanisms by Which Organisms Sense and Respond to Physical Properties of Surroundings and to Applied Mechanical Forces, Including Gravitational Force?
Impact and Rationale
Plants, microbes, and animals directly sense and respond to gravity as a so-called body force, and also respond to applied mechanical forces such as stretching (tensile forces or stresses) and compressing (compressive forces or stresses) at the cell and molecular levels. (See Figure 5-1.) Many cell types within these organisms can also exert mechanical force on their surroundings, with different molecular components acting as both force generators or force sensors; these mechanical effects are unrelated directly to gravity, particularly at biological length and force scales. In the space environment, it is possible to differentiate between direct and indirect gravity effects. On Earth, these effects are coupled, and thus it remains poorly understood how different environmental components interact with gravity sensing. Access to the space environment in LEO and beyond can reveal the mechanisms of force sensing that operate independently of or differentially in response to changes in gravitational force, in different organisms and at the level of cells and molecules. This mechanistic knowledge informs our understanding of phenomena taken for granted in a 1 g society, helps to clarify whether and when those phenomena will be replicated in different space environments, and may even elucidate why some biological processes are more rapid or energy efficient in space environments.
Animals and plants sense gravity directly by use of specialized sensors; all organisms sense mechanical stresses, due either to indirect effects of gravity or to other internal and external forces, in all of their cells and organs. Mechanosensing is fundamental to growth, development, gene expression, cellular growth, cellular differentiation, cell migration, bone modeling, muscle and vascular function, and endless other biological effects, and it is poorly understood. In some cases, the proteins that sense cell adhesion forces and the genes they control are known, but how cells detect and measure levels of stress (normalized force) and strain (normalized displacement) and respond to these forces is almost entirely unknown. The ability to experiment in space environments gives a unique opportunity to separate direct sensing of gravity from other mechanical effects. Little is known about how general environmental stresses—radiation (Boerma et al. 2005; Nagayama and Fukuei 2020), DNA damage, changes in atmospheric composition—alter the responses of cells to mechanical forces, and if the effects of these mechanical stresses are therefore mediated in part by otherphysical stresses.
Experiments on plant growth and development in space have been carried out for decades in pursuit of questions focused on the feasibility of using plants for food and regenerative life support functions. More recent studies have taken advantage of the spaceflight environment to pursue questions related to the fundamental processes of
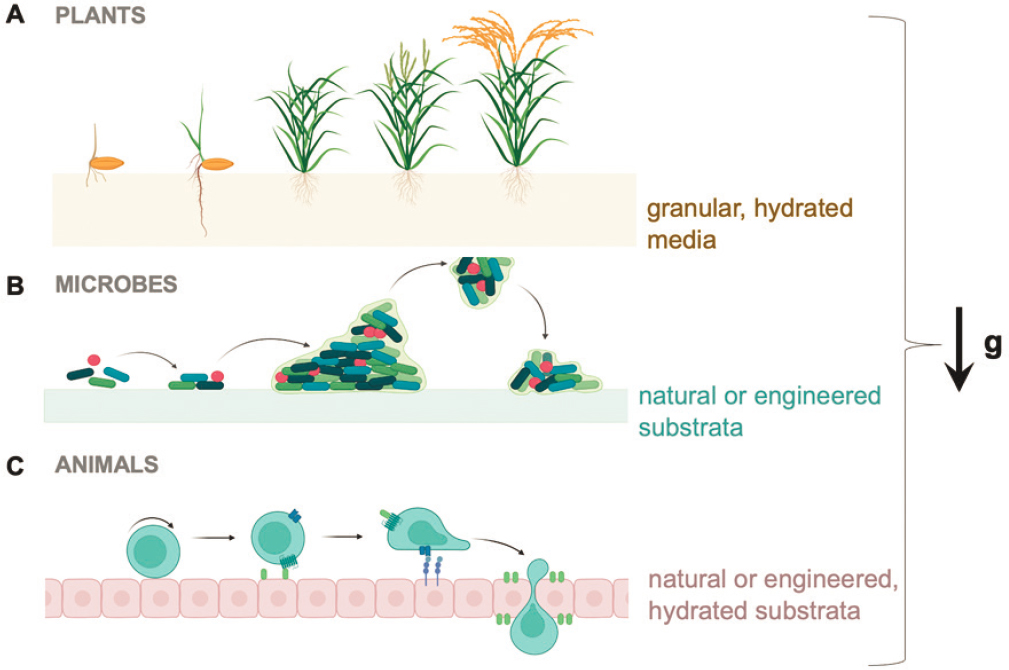
gravity and light perception and response pathways. Regardless of the motivation, access to the spaceflight environment has enabled questions regarding plant growth and development not possible on Earth. Experiments in low Earth orbit (LEO) have established that plants exhibit significant transcriptional reprogramming in response to the space environment, including differential gene expression (Barker et al. 2021; Chandler et al. 2020; Paul et al. 2017), as well as alterations in epigenetic states that control gene expression (Paul et al. 2021; Xu et al. 2021) and in alternative splicing of transcripts (Beisel et al. 2019). Because these studies compare space to ground controls, the responses may only be attributable in part to changes in gravity. The continued advancement of these questions will require additional hardware and more sophisticated analytical tools to begin to unravel the multitude of variables encountered by plants in the spaceflight environment.
While it is known that plants and animals can sense gravity directly—for example, by otoliths in the mammalian ear or by sedimentation of starch-filled plastids in specific plant cells—important aspects of the mechanisms remain unknown. Studies in the near-absence of gravity will enable the separation of gravitational forces from other mechanical forces necessary to understand the fundamental biological mechanisms by which gravity sensing occurs and the degree to which gravity sensors influence other mechanical responses. Because mechanosensing has a major role in guiding structural growth in biology, this understanding will have potential Earth translational
relevance to agriculture through plant morphogenesis under various environmental conditions and to human health through amelioration of the effects of aging, bone density decreases, and fibrosis.
Answering these questions requires experimentation in space as well as on Earth. Different gravity levels from micro- to hyper-gravity, including moon and martian levels, will enable distinction of graded responses and threshold effects of gravity and mechanical stresses whose sensation is affected by gravity, and will permit mechanical effects to be quantitated in the absence of gravitational load and of gravity sensing. This will allow a distinction to be made between the causal mechanisms of different effects, and their separate study. The known effects of the radiation environment on mechanosensing can only be partitioned between direct gravity effects and other mechanosensory effects in space, and the thresholds of response for any effect of gravity or gravitational loading can only be determined in orbit with appropriate centrifuges, and in the lunar and martian environments.
The proposed experimental approaches will answer this KSQ concerning the mechanisms and thresholds of gravity sensing and mechanical response in different organisms: plants, as critical for sustainable and climate-robust agriculture; and mammals including humans, as critical for understanding in vivo tissue de/regeneration and in vitro growth of cells as chemical production systems or as medicine. While not motivated primarily by space exploration risk mitigation, the fundamental research herein will also reduce the risks of space exploration by facilitating plant growth in space (and therefore providing critical nutrients such as vitamins for long-term space missions), or by pointing to methods to ameliorate muscle wasting, bone resorption, and therapeutic cell production and regenerative medicine for NASA-trained and civilian astronauts (Levine 2010).
Potential Research Areas
The experiments described in this subsection require access to the space environment for microgravity and radiation exposure, including access to centrifuge facilities in space for application of <1 g, as well as a means to capture response kinetics/behavior and preserve tissue for molecular analyses on Earth. The ability to resolve reporter gene expression and/or other physiological changes in real time during exposure to altered gravity treatments will allow understanding the dynamics of the responses, including -omics and epigenetics analyses. As a complement to the space experiments, more Earth-based hardware is required for preliminary experiments and ground controls, including centrifuges for hypergravity, clinostats and random positioning machines to mimic weightlessness (and to test by comparison to space responses), and equipment to replicate the vibrational effects encountered in the space environment as a control. Plant growth in space will require sealed growth chambers with controlled light, watering, atmosphere and ethylene scrubbing, and spectral analysis of plant health. Animal growth in space will require growth chambers suitable to the animal type. Last, a data depository and software approaches that allow a uniform analysis and presentation of data from space experiments and their ground controls is necessary for community utilization and analysis of results. This implies not only the maintenance and growth of GeneLab (see Finding 4-4), the current data repository, but also an increase in its analytical toolset, and coordination with other public databases of experimental results from genomic and other types of experiments. These potential research areas draw from different communities and require different technical capabilities in LEO and beyond and are thus organized by organism type without implication of ranking priority.
Plants
Mechanical and Gravitropic Mechanisms
Plants respond to gravity and to other mechanical forces. The gravitropic responses are thought to result from signals caused by sedimenting amyloplasts, starch-filled plastids that are found in gravity-sensing tissues and that move within cells when the gravity vector changes. The other responses, such as to stem bending during vibration or touch and meristem cell polarity that responds to local cell expansion or applied pressure (Hamant et al. 2008; Heisler et al. 2010), do not depend on gravity sensing through amyloplast sedimentation. They may (or may not), however, depend indirectly on gravity (as, for example, stem bending from wind, which results in shorter and thicker stems (Braam 2005), and may or may not require the additional force and stress distribution owing to gravity
acting on a stem that has been moved from the vertical). Some responses may depend independently on gravity and other mechanical stimuli, for example reaction wood (Groover 2016). Reaction wood forms asymmetrically in branches to reinforce them. It forms parallel to the gravity vector, when the branches are stressed by being bent. Does gravity determine the direction, and does bending stress determine the magnitude of the reaction in woody tissue, or do each contribute to both? The ability to remove or change the magnitude of the gravitational vector in the space environment will allow tests of these hypotheses regarding this long-term adaptation to altered gravity and non-gravitational mechanical forces.
The mechanism of gravity sensing in plants is poorly understood, and the transduction of gravity signals from the cells with amyloplasts is also only partially understood. The mechanisms by which bending is sensed, by which meristem cells polarize, and by which microtubules align to stress are largely unknown, despite active study. Microgravity and partial gravity, as assessed using centrifuges in LEO, and in the lunar environment, make possible a quantitative understanding of amyloplast sedimentation (e.g., by measuring sedimentation dynamics in partial gravity of different strengths). They enable the differential and combined effects of gravity and other mechanical forces to be recognized in all of the many mechanical responses of plants.
Decoupling of Gravity and Other Tropisms
The microgravity and partial gravity environments also allow for the decoupling of tropisms such as phototropism and gravitropism in plants. Plants respond to red light in a directional manner in microgravity but not in nominal 1 g on Earth (Kiss et al. 2012; Millar et al. 2010). Red-light experimentation was examined in combination with gravity-sensing thresholds (Kiss et al. 2000) made possible by the retired European Modular Cultivation System (EMCS), which had onboard centrifuges allowing for gravitational accelerations ranging from microgravity to reduced gravity to a 1 g control. These experiments demonstrated a robust positive phototropic curvature in response to unidirectional red illumination in the stems (i.e., hypocotyls of young seedlings) of plants that developed in microgravity. Time course studies showed a positive phototropic curvature relative to red light in microgravity and 0.1 g conditions, and the mean curvature was not significantly different between these treatments. In contrast, the response at 0.3 g was not significantly different from the response of the control (1 g), and red-light phototropism was attenuated in both. This range of reduced gravity (relative to Earth) is in the approximate range of gravity levels on the Moon (0.17 g) and Mars (0.38 g). These initial results suggest that lunar gravity may pose issues for plant growth, while martian gravity may be adequate; they also raise the question of a sensing mechanism that creates the threshold effect. The testing of such hypotheses will be enabled by the in situ research in the spaceflight environments of the ISS, Gateway, and lunar surface, and eventually martian surface.
Root Growth and Response to Resistant Substrates
Similar to the decoupling of the phototropic response from gravitropism, a comparison of thigmotropic responses under 1 g, 0.17 g, 0.38 g, and microgravity can show plant response to resistant substrates. For example, if regolith is used as a growth substrate versus a hydroponics or aeroponics approach, is there a substrate density that is not permissible for plant growth? Will a plant under partial gravity behave like a plant in microgravity when it encounters a surface it cannot penetrate? Will the root grow without direction, and will lighting need to be used to promote a negative phototropic response to encourage root growth into substrate (Scherer and Pietrzyk 2014)? Can plant tropisms and plant development in the spaceflight environment be explained in the context of mechanosensitive channels? These channels have been implicated in the perception of important mechanical stimuli such as osmotic pressure, touch, gravity, and pathogenic invasion (Basu and Haswell 2017).
Transcriptional Responses to Spaceflight and Partial Gravity
Plant transcription and epigenetic marks are altered in the space environment. Some of these changes are very likely owing to gravity alterations in space and to indirect effects of gravitational load on mechanosensing. Others may be owing to gravity effects on the plants’ external environment (such as alterations in the movement of water at the roots, or the effects of low gravity on convection and therefore gas exchange at leaf surfaces). A few studies utilizing an onboard centrifuge have focused on gravitational alterations, including interrogation
of a dose-dependent response to fractional gravity as well comparisons of transcriptional profiles in response to microgravity, lunar and martian gravity. The latter experiments have highlighted the potential modulation of the transcriptional response to partial gravity by red and blue light and indicate that more work is needed to further delineate this interaction. The expansion of available space environments to the lunar surface and work in weightless environments such as ISS and on the way to Mars would be greatly enabled by provision of centrifuges with sufficient capacity for growing whole plants at partial gravity, as a control at 1 g, and in hypergravity. It is essential that such gene expression studies under partial gravity are expanded to include additional species of plants (beyond Arabidopsis), and that future work assess the combined effects of gravity, light, space radiation, and the atmosphere in space vehicles such as planned for lunar and martian exploration.
Animals and Unicellular Organisms
Fluid Regulation in Vascular and Extravascular Spaces
The regulation of body fluid is profoundly different in microgravity compared to Earth. On Earth, the fluids in the body are pulled down toward the feet by gravity, and the fluids are returned back to the heart by the activity of muscles. In the microgravity environment of space, fluid accumulates around the head and the chest. In addition, rapid change in total blood volume, plasma volume, and red blood cell mass occur because the area to contain blood is decreased. The plasma volume and total blood volume decreases during the first hours in space and remain at a decreased level throughout spaceflight (Hargens and Richardson 2009; Hargens and Watenpaugh 1996).
Mechanical Effects on Cell Differentiation and Development
Animal cells respond strongly to mechanical stresses in their organization and development. For example, the internal organization of muscle cells, and their self-organization as they form muscle fibers, is a response to tension (Mao et al. 2022). Tension-driven multi-scale self-organization is also observed in muscle fibers grown in vitro from human-induced pluripotent stem cells (hiPSCs). Even in cells that are not considered highly contractile (such as muscle cells) or migratory (such as fibroblasts during wound healing), it is now well established that many tissue cells and stem/progenitor cells exert force to maintain adhesion with surrounding cells, extracellular matrix (ECM), or other materials encountered in experiments in vitro or implants in vivo.
Gravitational forces also control animal cell behavior and function. Cell growth and differentiation respond to altered gravity through reduced response to epidermal growth factor (EGF) (de Groot et al. 1991). In addition, intercellular EGF and PKC signaling is altered in microgravity (Boonstra 1999). This emphasizes the need for studies of whole organisms and multi-organ chips, as well as single organoids and isolated cells in culture.
Cells respond to microgravity through cytoskeletal reorganization and tension on ECM components (Hughes-Fulford 2003; Wu et al. 2022). Cell–cell interactions are likely impacted by the cytoskeletal reorganization. Changes in direct interactions between the cytoskeleton (CSK) and nuclear membrane involving the LINC (linker of nucleoskeleton and cytoskeleton) complex have been reported (Neelam et al. 2020). CSK reorganization may differ depending on the duration of exposure to both short- and long-term altered gravity.
Inflammatory factors also likely play signaling roles. Mechanosensitive endothelial cells show decreased secretion of cytokines in response to microgravity (Li et al. 2018), although many studies in other cell types show no response or increases (Ludtka et al. 2021). Given the roles of cytokines in wound healing and immunological health, more studies in both simulated and actual microgravity are warranted.
Nuclear Mechanotransduction
Nuclear mechanotransduction, or signal transduction transmitted within the cell and over the nuclear membrane to effect genetic expression, is a rapidly growing field of study and would provide an explanation for how individual cells might detect gravitational changes (Discher et al. 2017; Maurer and Lammerding 2019). Mechanotransduction at the cellular level has been implicated in a wide array of cellular processes such as proliferation and apoptosis, and in diseases such as cardiomyopathy and muscular dystrophy (Jaalouk and Lammerding 2009) as well as bone growth and remodeling (Moosavi et al. 2021). Understanding the changes in gene expression in
response to altered gravities would suggest mechanisms that could be used to develop space-specific countermeasures as well as Earth-based disease therapies.
Bacterial Mechanosensing
Bacteria are prokaryotic cellular organisms that share some structural and mechanistic features with cells in plant and animal systems. The roles and mechanisms of bacterial mechanosensing are understudied (Gordon and Wang 2019), leaving considerable scope for studies of the mechanisms of how mechanosensing works for these prokaryotic microbes in space environments, where fluid flow and consequently shear forces experienced by bacteria are expected to be altered by gravitational effects. Mechanosensing in bacteria is known to exist—in particular, in the formation of biofilms. The shear force experienced by bacteria after they transition from free-swimming to substrate-attached, and shear forces generated by bacterial motility on stiff substrates, can substantially change bacterial behavior and metabolite levels (Araújo et al. 2016). Flow velocity affects the characteristics of Pseudomonas fluorescens biofilms, and shear stress affects growth, adhesion, and biofilm formation of Pseudomonas aeruginosa with antibiotic-induced morphological changes (Fonseca and Sousa 2007). Long-term bacterial adaptation to gravity changes will point to the underlying cellular mechanisms and signaling pathways that bacteria use to sense their physical environments and can provide insights into how cells in multi-cellular organisms also respond to gravity changes at the cellular level.
Finding 5-2: Access to microgravity and other aspects of the space environment is required to truly understand how microbes, plants, and animals respond to body forces and applied forces at the molecular level.
Question 9: What Are the Fundamental Principles That Organize the Structure and Functionality of Materials, Including But Not Limited to Soft and Active Matter?
Impact and Rationale
On Earth, transport is mediated by gravity (in addition to other applied forces). Gravity is the driving force for fluid flow—even on small scales such as when considering capillarity, surface tension, and Coulombic forces that are balanced by gravity. On Earth, the weight of a buoyant material is balanced by the weight of the displaced fluid. However, in microgravity, surface tension forces dominate in both cases. With respect to heat transfer, natural convection is driven by fluid motion owing to the differences in the relative densities of the fluids. Warmer fluids of lower density will rise, while cooler fluids with higher density will fall owing to gravity. In microgravity, differences in fluid density will not cause what is considered on Earth to be natural convection; this lack of natural convection affects temperature, constituent concentrations, and distributions of both temperature and concentration.
In the terrestrial environment, gravity obscures important forces and phenomena because of buoyant effects that govern most processes that involve the transport of mass, energy, and species. Vertical motion occurs owing to density differences that promote mixing within phases that disrupt the dynamics of reaction fronts or limit the effective range of other forces (i.e., both surface and body/volumetric forces), such as surface tension, electromotive forces, magnetic induction, and so on. There are two common types of buoyancy-driven motion: one is driven by a density difference between phases, such as a vapor bubble rising through a liquid, and the other is driven by the density difference of a reaction front like a flame or plasma discharge within the same phase and has been typified by the contrast in candle flames in normal and microgravity. As such, new discoveries have been found like the experimental evidence that first confirmed the existence of “cool flames” (Nayagam et al. 2012) and “warm flames.” Flotation, seen as the bubble rising through the liquid, or conversely, sedimentation, limits the observation time in a static situation or equilibrium condition in a terrestrial environment. In addition, the object ceases motion when it encounters an obstruction such as a container boundary, and the structure of agglomeration is primarily affected by the physical boundary, as opposed to other forces and neighboring objects.
Natural convection is the motion owing to the density variation within a single phase and is typically attributed to temperature or concentration gradients. This motion promotes mixing and overwhelms diffusion of chemical reactants and products and disrupts the range and impact of the dynamics of reaction fronts and the charge effects.
This mixing alters chemical reaction rates, heat transfer, and mass transfer across surface and interfaces. In normal gravity, the mass of fluid impacts both itself and its contents. The fluid “weight” distorts deformable volumes such as bubbles, droplets, and cells. The three-dimensional symmetry of such spherical objects provides experimental data to validate simplified symmetrically based models for heat and mass transfer and the limiting case of the minimal surface area to volume ratio.
Access to the space environment is essential here, because in a wide range of applications, theories and models of complex fluid and chemical behaviors ignore, for the sake of simplicity, the gravity terms in the governing equations. Experiments in terrestrial laboratories, however, are (with few exceptions) all conducted in the presence of Earth’s gravitational field. This gravitational field can obscure important phenomena and dominate the problem of interest rendering the direct comparison of experiment and theory/model difficult or even impossible. Experimentation in the low-gravity environment then achieves the idealized condition of a negligible gravitational force term and buoyancy-induced flow, thus providing an experimental capability that is nearly impossible in terrestrial laboratories (except for the reduced gravity facilities on Earth such as drop towers and aircraft flying parabolic trajectories), and allows investigation of the influence of other factors on determining the fluid flow, chemical reactions, vibrationally and electronically excited states, and phase change processes.
The transport of mass and energy affects both the structure and composition of all phases of matter (as well as phase change phenomena). While it is possible to impose larger local accelerations using centrifuges to assess the effect of gravity, it is not possible to ascertain these other factors (such as buoyancy driven flows, which will not only reposition material elements but also drive the flow around these elements, thus exposing them to varying conditions). The momentum, heat, and mass transfer are coupled within each phase, while the discontinuities at the interface between phases and across the reaction wavefronts can make the coupled and non-linear analyses of such scenarios more complicated.
In general, experiments conducted in space environments to date have been with relatively benign materials under a narrow band of conditions owing to safety concerns to both the crew and spacecraft. While there is a tendency to lump many chemical compounds as either “liquids,” “solids,” or “gases,” and focus solely on traditional transport and thermodynamic properties (such as density, viscosity, specific heat capacity, thermal conductivity, surface tension, contact angle, latent heat, etc.), there are other factors that can significantly alter their behavior. For example, liquid hydrogen, which has an extremely low surface tension and density compared to water, has the largest flammability. Most aqueous solutions tend to have properties that are similar to water, but minute amounts of sodium lauryl sulfate or other surfactants dramatically change the surface tension and wetting characteristics. Some perfluorofluids are highly nonpolar and will not dissolve many organic and ionic compounds but absorb large quantities of gases. Experiments could be conducted considering different test fluids, varying concentrations of additives, and different configurations (of flow conditions, sample containers, and geometries) to improve the statistics. The varying concentrations of additives can serve as precursors for forming nanoparticles and colloidal solutions from in situ chemical reactions where these in situ chemical reactions can be triggered by external stimuli (such as heating, light, radiation, ultrasonication, sol-gel reactions, electro-magnetic induction, subjecting to microwave radiation, etc.).
While multi-phase flows have been viewed within the NASA biological and physical sciences (BPS) and engineering communities principally as gas–liquid or vapor–liquid flows, the definition needs to be extended to include the solid phase, immiscible liquids, and emulsion. The lack of gravity limits the settling rate of solids, and this prolonged suspension permits other forces and reactions to occur. Single and double “entity” or multiphase systems (such as bubbles, droplets, particles, etc.) need to be investigated in order to assess the impact of imposed fields on the ability to induce motion (enhance transport of mass, momentum, and energy), change phase, merge (enhanced mixing), and react with its host medium and the system boundaries. Ultimately, the experiment measurements and modeling need to be extended into systems that contain not just a huge quantity of particles but also a wider dispersion of sizes as well as heterogeneous interfaces in order to gather population behaviors and to identify the stability envelope of such systems.
For example, phase change materials are considered to be attractive for thermal energy storage by leveraging their high values of latent heat of fusion. However, salt-hydrates suffer from multiple issues that compromise their reliability, especially for initiating nucleation and during the solidification processes. This can include diffusion
limited transport, chemical stratification, and so on. These effects can become acute under varying levels of gravity (that are nearly impossible to implement and study in Earth-normal gravity environments) as well as for different sample-size conditions and different configurations of heat exchangers (involving different flow conditions of the heat transfer fluids, geometries and containment architectures). Containment architectures within the heat exchangers can involve fins, foams (metal or carbon foams), acoustic/ultrasonic agitation (for enhanced mixing), nucleation promoters/additives, incorporation of gelling agents, and so on. The power ratings if such heat exchanges are used for thermal energy storage can vary significantly, owing to absence of natural convection, both during melting and solidification cycles. This cycling is akin to charging and discharging cycling, whether for thermal management applications for electronics chip cooling or for sustainability applications for reduced water usage in power production.
Last, materials and mixtures governed by weaker internal interactions can exhibit both liquid-like and solid-like characteristics for which the organizing principles of structure and phase change stability remain incompletely understood. Such materials that can flow readily under gravitational force (i.e., are easily deformable under body forces or applied forces, whether reversibly or not) are referred to as soft matter. Such materials that can self-assemble in response to fields or stimuli, or can generate force against surrounding environments, are referred to as active matter. (See Figure 5-2.) For such material types, access to the microgravity feature of the space environment is critical to gain such knowledge about how the functional units or “particles” interact to provide and change structure in response to applied stimuli. Because the interparticle forces are rather weak forces, gravitational stresses limit the size of the crystallites to tens of unit cells per side; in space environments, larger assemblies and masses suitable for fundamental experiments are possible. Moreover, many soft matter systems are microscopic, colloidal, and suspensions of particles in fluids. They are difficult to density match and, in a terrestrial environment, they sediment, which restricts experiments to two dimensions. Opening the three-dimensional world to these materials with microgravity allows a much greater variety of phenomena, types of organization, and different materials and processing. Furthermore, in terrestrial gravity the driving temperature, concentration, and magnetic, electric,
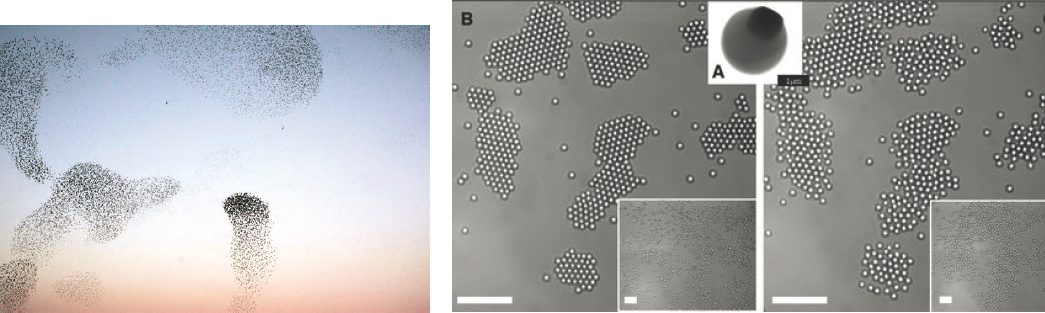
and light fields create density gradients and produce flows that interfere with the basic phenomena of interest. Microgravity allows for isolation of the fundamental interactions and dissection of the phenomena.
There remain many important basic questions about soft matter in equilibrium. Such materials are constructed from building blocks that have internal degrees of freedom and internal sources of power and are different from conventional materials where the building blocks (atoms or simple molecules) and their interactions are well described. These engineered materials can exhibit a much wider set of properties and functionalities, and approach the functionality of natural, living materials. Rather than simple polymers, emulsions, colloids, and granular matter that interact through conventional forces, researchers have produced solid and liquid particles that interact through a host of forces. These now include DNA hybridization that can activate the motion of functionalized colloids and emulsions, biomolecules, proteins, and enzymes; entropically driven shape-dependent properties (lock and key colloids) that enable design of arbitrary shapes with artificial molecular motors that power the motions (translation and rotation) of particles; and nanocomposite tectons that self-assemble to create structurally distinct phases of matter (Zhang et al. 2016). Using the new forces and particles, great headway was made in Earth-based experiments over the past decade, including development of colloidal soft matter as photonic crystals and in the modeling and engineering of active soft matter. (See Figure 5-2.) These new tools will give rise to novel materials with exciting properties such as sensing and repair, shape-shifting, self-healing, and self-replication. But there is much we do not know about how these passive and active soft materials interact with the space environment; these unknowns are part of stated Grand Challenges in Soft Matter Science (Mezzenga 2021).
Aside from the processing and materials aspects that are established under steady-state or equilibrium conditions, the new building blocks allow production of dynamical systems for testing some of the most basic aspects of systems during transients and far-from-equilibrium (see Question 10). Using the design capabilities of soft and active matter, model systems can and have been made to test the role of entropy and entropy production and how it relates to where work can be extracted. Such techniques developed in soft/active matter have further been applied to biological systems. The quantitative analysis of these experiments is only possible owing to advances in machine learning, artificial intelligence, big data, and simulations. These developments affect not only the way data are currently analyzed but also how experiments are being conceived and executed.
Finding 5-3: Preceding decades of microgravity research have shown the promise of using the reduced-gravity environment to provide transformational change by identifying new states of matter, new physical phenomena, and emergent material properties.
Potential Research Areas
Chemical Kinetics and Combustion Processes
Experiments in microgravity have provided unprecedented insights into chemical kinetic phenomena, highlighting the importance of radiative loss in determining flammability limits, new steady-state regimes of low-temperature and intermediate-temperature fuel burning phenomena (cool and warm flames) and insights into sooting and the formation of large soot agglomerates that has enormous impacts on understanding the effects of wildfires on the environment and applications to material synthesis. Microgravity experiments also demonstrated that electric field and charges (ions and electrons) can also affect the energy transfer, reactions, and flow motion. However, large buoyant flows present in terrestrial laboratories prevent the attainment of the low strain environment necessary to study systems where flame structures or reaction wave fronts are determined by chemical kinetics and motion of charged molecules and particles. Fundamental experiments are necessary for developing predictive tools for chemical kinetics over a range of environments and fuels including biofuels, e-fuels, and low-carbon fuels.
Microgravity studies have shown that large soot agglomerates (super agglomerates) are preferentially formed in microgravity flames where, in the absence of buoyancy, the long residence time necessary for the formation of these structures is easily attained. Reduced-gravity studies of soot formation, including the formation of super-agglomerates, could further elucidate this phenomenon. The low stretch/strain environment is readily attained in reduced gravity studies in the absence of the buoyant flow present in all terrestrial studies. This environment
provides the strictest test of chemical kinetic mechanisms, as the flame structure limits are kinetically controlled and not determined by the flow environment, providing insights into the fundamental physics of the processes involved.
Multi-Component Multi-Phase Behavior
Spaceflight systems utilize both single-component fluids such as propellants and multi-component fluids such as providing water and fertilizers to plants. However, many space experiments have selected single-component fluids for investigation, thus avoiding identifying and quantifying the effect of these components on the overall behavior.
Compared to a terrestrial laboratory, in a space environment, trace amounts of other components may affect the behavior in terms of contact line motion, coalescence, agglomeration and solidification, and interfacial heat and mass transfer. Experiments could quantify the scale of behaviors relative to the concentration of solutes, to understand the limits to which pure fluid analogy is sufficient with regard to “infinite dilution” assumptions. Another effect that can be clearly addressed in space environments is how the imposition of external fields—that is, electrical, magnetic, and so on—affects the phase change process, the orientation, motion, and separation of components and phases.
Effects of the Space Environment on Transport and Materials Synthesis
These microgravity-driven differences in transport phenomena can enable synthesis and fabrication of novel multi-component materials and structures. Additionally, microgravity enables containerless synthesis (Amselem 2019; Broutin et al. 1997) and processing. With respect to material synthesis and processing, the following narrower research areas could be considered:
- Effects of gravity on nucleation, growth, phase transformation, and the evolution of microstructure. While these questions have been probed for some single-component systems, further investigation is warranted of microstructure and its evolution for complex, multi-component materials fabricated in space. Furthermore, even in single-component systems, novel insights are emerging, owing to unique methods of processing and measuring these materials that are enabled by the space environment (Bracker et al. 2020).
- New materials synthesis routes enabled by the space environment. The space environment affords different processing methods and conditions than terrestrial environments. An area of future investigation may be focused on synthesis routes that are impossible/impractical on Earth but can be achieved in a space environment (e.g., nanoparticles formed in situ from additives when specimens are subjected to extraterrestrial radiation).
- Effects of the space environment on complex crystal structures. Possible areas of inquiry could include polymorphism and order–disorder transitions. Crystal polymorphism refers to the same molecules crystallizing in different ways, which can affect properties and performance. Microgravity affects dominating forces and transport phenomena, which may enable synthesis of new crystalline polymorphs. Most prior work on crystal polymorphism in microgravity has looked at a single protein (Amselem 2019), which could provide a foundation for more complex crystalline structures. Order–disorder transitions can be probed under microgravity conditions, leading to greater knowledge and measurement of the atomic interactions that lead to order–disorder in a system.
- Special opportunities in space for the manufacture of drugs and expensive chemicals. The unique environment afforded by space may enable synthesis and processing of molecules/macromolecules that cannot be practically manufactured on Earth. Possible advantages enabled by space include containerless manufacturing, manufacturing in water-free environments (for compounds with low water stability), and crystal polymorphism (Weber 2010).
- Glasses and other amorphous materials made in space. Amorphous materials could be cooled sufficiently fast to avoid nucleation and crystallization. Containerless processing avoids nucleation owing to contamination of the container surface. However, microgravity reduces heat transfer via natural convection, which may lower cooling rates if radiation is not significant. Fabrication of more complex, multi-component amorphous systems requires understanding how these materials differ from those made on Earth.
- Material behavior during processing in reduced gravity. Processing within this context may include extraction, refinement, manufacturing, and reuse/recycling. When gravity does not dominate, other forces may become more important, as discussed above. Identification of the governing physics and chemistry in reduced gravity will also enable measurement of these forces with resolution that cannot be achieved on Earth.
- Critical heat flux conditions. Conditions at fixed, transitory, and oscillatory pressure conditions could be examined to assess dampening and acoustic-like effects, with focus on changes owing to space environment including microgravity. For example, as the pressure is increased in a boiling system, the saturation temperature and heat of evaporation increase at a modest rate, but the vapor density varies linearly. Thus, the transition to film boiling is delayed because the bubbles accommodate higher rates of vapor generation, resulting in a peak in critical heat flux values with increase in operating pressure. However, at higher pressures, this behavior reverses, owing to the drastic reduction in surface tension near the critical point.
- Fluid compressibility. Given the lack of natural convection in microgravity, compressibility effects, especially at higher pressures, could be examined because many investigations have occurred at pressures near atmospheric. Solubilities, chemical reaction rates, and heat and mass transfer rates change at higher pressures (e.g., near critical pressures). At pressures approaching and above the critical point of the fluid, reductions in diffusive timescales governing thermal, mass, and momentum transport occur as well as changes in solubilities that impact chemical reaction rates and rates of phase transitions (e.g., phase change heat transfer).
- Phase change processes. Phase change processes could include studies that can take advantage of a quiescent environment that promotes both mass and thermal diffusion that may lead to different morphologies of bubble nucleation distribution as well as growth and departure. This includes solid–liquid and solid–vapor transitions as well as solid-state transitions. Materials that are elastic and compressible could be tested in microgravity, as the sag introduced by gravity does not complicate the analysis of the behavior. Where possible, the characteristics of extraterrestrial media such as lunar regolith could be measured and assessed for its processability.
Effects of the Space Environment on Material Properties
Key structural, physical, and functional properties of materials can change, owing to environmental exposure. On Earth, one observes this in everyday objects like rusting metal infrastructure or contact lenses that dry out when not stored in saline solution. Space offers environments unlike terrestrial ones, which can be hostile to Earth-familiar material stability owing to different (or no) gaseous environments, temperatures and temperature ranges, extraterrestrial radiation environments, electromagnetic fields, and varying levels of gravitation. While the role of different levels of gravity in material synthesis and processing and the science they enable are discussed in detail above, these other differences may also fundamentally change material properties and associated utility of materials in space. (See Figure 5-3A.) With respect to material properties in space, the following narrower research areas could be considered:
- Effects of the ambient environment on properties of materials in space. This includes considerations such as temperature, vacuum versus gas pressure, extraterrestrial radiation, and oxidizing versus reducing conditions.
- Combined measurements including properties. For experiments on materials in the space environment, interpretation of the results depends critically on the properties of the sample. For many classes of these experiments, the properties of the sample can be measured in situ with the same apparatus. This approach gives not only accurate properties of the material, but also accurate properties of that sample at the time of the experiment.
Colloidal Phase Behavior
Dispersed networks of stimuli-responsive colloids, polymers, and proteins can be actively driven to undergo phase transformations or maintain metastable states that produce metamaterials with unusual physical properties
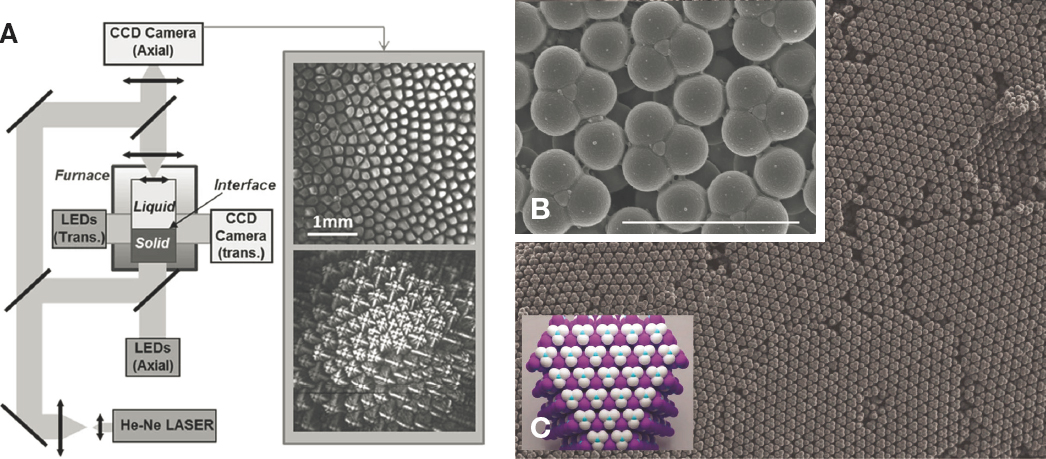
(Aubret et al. 2018; Soni et al. 2019; Spellings et al. 2015). The space environment enables exploration of model colloidal systems with diminished gravity and pressure that limits buoyancy-driven convection, sedimentation, hydrostatic pressure gradients, and liquid film drainage, and can stratify density gradients. The effects of increased radiation and temperature fluctuations can also play significant roles in colloidal behavior. In this environment, a wide range of phase behavior of fragile systems near and far from equilibrium can be effectively probed, including syneresis, coacervation, jamming, and bicontinuous particle-stabilized emulsion (bijel) formation.
Effects of Anisotropy and Self-Motility in Phase Separation
The time-reversal symmetry of low Reynolds number environments can be subverted by the spatiotemporal asymmetry of active colloids (Chen and Santangelo 2018; Chen et al. 2021), which can induce liquid–liquid and liquid–gel phase separation. New orderings of soft matter have recently been reported that have intriguing properties, such as the ability to output power rather than dissipating it. The reduced gravity of the space environment may enable the discovery of even more exotic forms of weakly bound network materials, phase transformations, and new phenomena.
Plug-and-Play Assembly Methods for Soft Materials
The ability to destroy and reassemble soft materials into structures with tunable properties has been proposed; however, design rules and methods to assemble these materials and structures in the space environment have not yet been developed.
Active Matter
For both passive and active soft matter systems, shape, internal structure, and distribution of electrical charges, chemical activity, flow, and other external stimuli affect the overall structure and properties of intermediate and final products formed through self-assembly processes. In addition, for both homogeneous and heterogeneous active systems, dynamics of individual building blocks can vary within a population and alter self-association, adding significant complexity to dynamics that give rise to bulk soft materials, which in some cases can possess properties not observed in natural materials (Mallory et al. 2018).
Actuation of Colloidal Suspensions to Form Scalable Metamaterials with Tunable Physiochemical Properties
Relatively weak external fields, including magnetic, acoustic, and phoretic, have shown great potential to drive colloidal suspensions into reconfigurable states with desired properties. (See, e.g., Figure 5-3B,C.) However, it is yet to be determined how these transmitted signals can be used to form, hold, and fix weak colloidal structures that would be otherwise destroyed by gravitational forces on Earth.
Such studies, along with ground-based counterpart baseline experiments at 1 g, could enable the first well-controlled measurements of the forces driving self-assembly from the independent effects of gravity, buoyancy driven flows, and hydrostatic pressure. One could construct phase diagrams for concentrated suspensions of anisotropic active matter with and without the presence of external fields (hydrodynamic, electromagnetic, pneumatic, and acoustic). That knowledge could in turn enable design principles for materials enabling multi-scale soft robotics, and micro- and nano-scale colloidal machines that are mobile, reconfigurable, sensing, and capable of manipulating their space environment.
Material Synthesis and Processing
The reduced gravity environment of space and the lunar surface leads to greater importance of other forces (e.g., capillary, electromagnetic, van der Waals, thermophoretic, and Coulombic) and also fundamentally changes dominating transport phenomena. Understanding the new balance of forces and how they affect energy, heat, mass, and momentum transfer in reduced gravity is critical to controlling synthesis and processing across levels of gravitational forces and can enable creation of new multi-component materials (e.g., alloys, organic molecules/macromolecules, including those with complex secondary structures, and composites), and structures ranging from nanomaterials to meter-scale systems, and chemical synthesis. The experiments necessary to generate this new knowledge require the space environment.
Question 10: What Are the Fundamental Laws That Govern the Behavior of Systems That Are Far from Equilibrium?
Impact and Rationale
All systems undergoing change, whether mass and heat transport or chemical reaction and phase formation, are out of thermodynamic equilibrium; systems tend to respond to bring them closer to equilibrium. When the deviation from equilibrium is small, conventional kinetics and statistical mechanics can provide theoretical underpinning. However, when systems are very far from equilibrium, such formalisms become tenuous and there is need for new theory, chemistry, and physics. The opportunity for well-designed space-based experiments is critical to identify and validate the laws that govern material behavior when systems are far from equilibrium. (See Figure 5-4.) Equilibrium thermodynamics is governed by one basic principle from which all else derives: in equilibrium, entropy (a measure of the number of equivalent equilibrium configurations in a system) is maximized. Non-equilibrium phenomena have no such unifying principle, challenging our ability to understand, predict, and control the behavior of materials that are far-from-equilibrium.
Two of the fundamental laws of nature are that matter can neither be created nor destroyed, and that energy can neither be created nor destroyed. Both laws assert a balance in both the total amount of matter and energy, even though the amounts of specific molecular arrangements or type of energy can transition (kinetic versus potential, electrical versus thermal, etc.). Thermodynamics has used this balance or equilibrium successfully for predicting
potential results and behaviors where the deviations from equilibrium are small. However, some conditions may be a case of “quasi-equilibrium,” whereby small perturbations lead to significant changes such as a docile mixture of highly reactive chemicals that require an activation energy to initiate the event. In addition, technological advances have occurred in a range of applications that use systems/processes where the deviation from equilibrium is large enough that methods to predict results based on equilibrium fail because other mechanisms supersede the slow and gradual changes that form the basis for an equilibrium approach. These mechanisms rely on mass and energy that are transported into a region, upsetting the local equilibrium state, and can extend into a macroscopic scale. Even energy storage materials such as those within batteries are subject to such far-from-equilibrium (FFE) scenarios during rapid, cyclic charging and discharging across material interfaces.
The microgravity environment provides nearly uniform hydrostatic pressure and a significant reduction in natural convection, which together enable research in high-pressure and non-equilibrium phenomena. These variations can affect thermophysical properties, reaction kinetics, and material solubility, as these are affected by the dominance of strong intermolecular forces and non-elastic multi-body collision processes. Descriptions of fluid behavior in the vicinity of the critical point critically rely on an assumption of equilibrium throughout the fluid domain, or that deviations from equilibrium are minimal (from a system-wide predictive viewpoint).
Indeed, access to the space environment, including sustained microgravity conditions, is required to advance this KSQ. Technologies are increasingly reliant on computational models to shorten development time; optimize designs; and reduce costs relative to more traditional strategies involving building prototypes, testing, and redesigning philosophies. This expectation requires a predictive capability from models that need significant refinement and sophistication. In some cases, predictive models are unavailable because of a lack of fundamental data on thermophysical properties and chemical kinetic processes and in other cases where conventional theories (based on equilibrium thermodynamics) do not apply. For example, rapid solidification of metals often leads to FFE conditions with consequences to the resulting microstructure and properties. In stainless steels, a metastable crystal structure forms with a composition different than predicted at equilibrium because the solidification velocity overwhelms the diffusion necessary to reach local equilibrium. Furthermore, the rapid solidification causes a large concentration of defects in the metastable crystal, observable as broader detection peaks in Figure 5-4. Access to reduced gravity for experiments in the space environment and many ground facilities over the past decade provided wider access to stirring and undercooling parameters. This in turn enabled theoretical modeling to support discovery of an additional energy term that can now predict this unanticipated effect on a well-known material. Space environment is required of such discoveries, but the practical impacts are realized first on Earth in terms of quality and properties of metallic alloys cast for many structural infrastructure applications.
The aforementioned studies have identified new states of matter and new physical phenomena that have had profound impacts on their respective disciplines (e.g., see Chapters 2 and citations in Figure 5-4); these discoveries can be fueled by a laboratory environment where the gravitational body force is essentially eliminated. Addressing this KSQ extends our fundamental knowledge of how all classes of materials change form and phase; such knowledge will enable predictive modeling and insights. History indicates that such fundamental understanding of material physics beyond that predicted from current thermodynamic laws will confer human impact in ways that are not yet imagined; our ages are often named by the materials that can be processed for human needs. Anticipated practical applications include processing and manufacturing of structures and machines and potentially creating materials that cannot be fabricated similarly on Earth.
Potential Research Areas
Chemically Reacting Systems (Gases) at High Pressure
For fluids at high pressure and rates of change in pressure, intermolecular forces are enhanced as the pressure increases and the molecule mean free paths are reduced. As a result, the likelihood of multi-body collisions is increased. Additionally, strong intermolecular forces contribute to increasing the complexity and dynamics of the chemical kinetics. Moreover, radiative transport becomes increasingly complex as gray-gas (optically thin) assumptions may fail.
This consideration extends to boiling: not only does the distribution of the surface tension forces at the liquid–vapor interface change, but also the solubility of noncondensable gases in the liquid phase changes (which affects the dynamics of bubble nucleation, growth, and departure). The mass transfer in both phases is impacted also

because of the increased probability of multi-component molecular interactions that can retard reactions because of the increased interaction between different chemical species.
Non-Equilibria Phenomena in Complex Fluids
Increasingly, new and emerging technologies involve phases of matter not easily classified into solid, liquid, and gas categories: “squishy” soft matter (with some examples also called complex fluids), as well as transcritical phenomena involving fluids near and exceeding the critical point and plasmas. These systems are in states characterized by weak interparticle interactions (as discussed in Question 9, at equilibrium on Earth), and the space environment can thus push such matter toward FFE behavior that cannot be predicted. Outcomes of such research
are expected to be most relevant in engines moving to high pressure; advanced engine concepts—kinetically controlled engines; and non-equilibrium, plasma-assisted combustion and chemical manufacturing.
High-Pressure Transcritical Research
Experiments are necessary to provide fundamental properties and develop predictive models for single- and multi-component mixtures at high and transcritical pressures. An important research goal is to develop a predictive capability for reacting systems at high-pressure conditions, given the importance of three-body or multi-body collisional reactions and the increased importance of intermolecular force and radiative transport. It is important to consider (and in some cases, reassess) the fundamental laws that govern the phase equilibrium, phase interfaces, and heat and mass transport at high-pressure conditions extending beyond the critical points of the constituent fluids.
Scaling Predictions
This term has many connotations distinct and relevant to reduced gravity, ranging from length scales, population size or concentration, and phenomenological to system behavior. One such connotation involves the “jump” conditions of the temperature and concentration profiles at the interface in a phase change or across a chemical reaction wavefront process. Of practical interest to many applications are the controlling mechanisms in nonequilibrium microchannel flows where traditional continuum fluid mechanics do not adequately define the jump or slip boundary conditions behavior. Another scaling-related issue arises as the effects of forces on single- or two-body events are defined. The impact of multi-body interactions remains unclear—collisions and interference can either diminish or punctuate the effect of those forces.
For certain processes such as interfacial bonding among materials, it is important to determine if their response to acceleration or gravity is linear. The scale range here extends from zero gravity potentially to supergravity (giant-planet environment) and includes partial gravity environment (lunar and martian surfaces). Bonding is a useful example of a process affected by the residual gravity level, and studies of bonding among dissimilar materials in distinct space environments will be informative.
Dryout and rewetting on localized heat transfer surfaces impacts the stable operation of a two-phase system, and again, the role of acceleration, gravity, and partial gravity needs elucidation, in combination with the effects of surface geometry, pinning edges and flow paths upon the two-phase system stability. Other mechanisms may exist for the preferential accumulation and release of phases within a multi-phase system, impacting the stable operation of that system.
Flow and Structure
Even though matter may flow, when subjected to activating events—matter will react to these events and reorganize into new structures; these range in length scale of order from the microscopic to long-range macroscopic architectures. Determining the relationship of the motion of suspended bodies when subjected to external fields as well as localized charges and shapes may be deterministic in terms of the final structure. These fundamental questions can be studied in several different types of matter, leveraging the unique space environment characteristics, including metallic alloys, organic soft matter, and composite materials governed by colloidal interactions.
In a microgravity or partial gravity environment, it is possible to conduct clean experiments to determine how much and what type of impetus could be applied to a system at or near equilibrium to initiate a rearrangement. These experiments will help to identify the factors that affect the behavior rate of the morphing, translating, and/or rotating, as well as determine if there are multiple “end-states,” and what determines which end-state is achieved. These end-states may be influenced by hysteresis and/or be “path dependent.” Experiments that can quantify this in materials described as undercooled liquids or glasses can also advance predictive understanding and modeling of metastability and phase separation, as well as kinetic limitations in phase transitions.
Such understanding will contribute to a unifying goal of “model” systems for which generalized laws for nonequilibrium phenomena can be developed. One example of such a generalized law could be a framework uniting classical hydrodynamic instabilities (e.g., Richtmyer-Meshkov instability) and their multi-phase analogs (in the case of Richtmyer-Meshkov instability, the analog would be shock-driven multi-phase instability).
Statistical Mechanics for Active, Particulate Materials
There has been significant research into colloid interactions in simulated microgravity and on the ISS; however, since the 2011 decadal survey there have been numerous reports of novel active colloids. These particulate systems, whether powered through fuel or fuel-free energy sources, can assemble into network materials with unusual bulk properties (Okutani 2002; Weber 2010) and behavior, including self-healing, jamming, odd viscosity, auxetic behavior, and allostery. Currently, it is difficult on Earth to experimentally identify, decouple, and precisely measure the specific forces interactions that lead to the observed bulk behavior. The space environment provides an ideal test-bed to explore active colloidal interactions beyond and among equilibrium states. The reduced gravity states of the space environment will enable the first answers to important yet seemingly basic questions about these materials, including whether steady-state flow can exist between two different non-equilibrium states. This space-specific advantage is because sedimentation owing to Earth’s gravity will be suppressed.
Question 11: What New Physics, Including Particle Physics, General Relativity, and Quantum Mechanics, Can Be Discovered with Experiments That Can Only Be Carried Out in Space?
Impact and Rationale
An intellectual crisis in fundamental physics provides an opportunity for space-based research. The 21st century brought forth this awareness of our collective limit in understanding fundamental physics—that is, our understanding of the physical laws governing matter, space, and time. On the one hand, the standard model (SM) of elementary particles and fields (Particle Data Group et al. 2022) has been extremely successful in predicting and explaining a plethora of physical phenomena. Also, with the discovery of the Higgs boson, all particles of the SM have been observed. At the same time, plentiful observational data on galactic and larger scales cannot be explained based on the observed matter content in the framework of the SM and “normal gravity” as described by the weak limit of general relativity. The prevailing view is that invisible “dark matter” (Figure 5-5) dominates the matter content of the universe (Bertone and Hooper 2018). Attempts to explain dark matter by simply “invisible” normal matter (i.e., faint stars, black holes, large planets, etc.) have failed—this possibility has been ruled out via detailed astronomical studies (Tisserand et al. 2007). In addition, observations indicate that the universe is expanding at an accelerating rate, an effect commonly attributed to “dark energy” (Frieman et al. 2008). A summary of all available data gives that only 4.93 percent of the matter-energy composition of the universe is “normal matter” (i.e., the SM particles) (Particle Data Group et al. 2022). Cold dark matter constitutes 26.5 percent, and the remaining 68.5 percent is dark energy, with both dark matter and dark energy of unknown origin (Particle Data Group et al. 2022).
Most dark matter searches to date look for a specific candidate, which has been seen as very promising from the perspective of theoretically favored extensions to the SM—that is, weakly interacting massive particles (WIMPs). However, despite much effort and advances in detectors, more than 2 decades of such direct WIMP dark matter searches have not yielded a discovery (Arcadi et al. 2018). As a result, the past decade has seen unprecedented effort in broadening the candidates for dark matter, extensive model building at all mass scales, and the design and implementation of numerous new types of ground-based detectors (Antypas et al. 2022; Bataglieri et al. 2017).
In addition to the dark matter and dark energy problem, the standard model fails to provide explanations for several other phenomena and issues. Examples include the hierarchy problem (why are the masses of known particles so much lighter than the fundamental energy scales of the unification of fundamental forces?); the matter–antimatter asymmetry problem (why is so much more matter than antimatter observed in the universe?); and the existence and origin of neutrino masses. Moreover, the class of beyond standard model (BSM) ideas involving supersymmetry predicted new particles within the observable range, which the Large Hadron Collider (LHC) so far has not detected (Canepa 2019). This presents a further crisis, now for the leading BSM theories.
Answering this KSQ, even partially, over the coming decade of space-enabled BPS research will help address and inform underlying subquestions, including: How are gravity and quantum mechanics connected? Does general relativity hold in extreme regimes, such as near or inside black holes? Are fundamental constants actually constant?
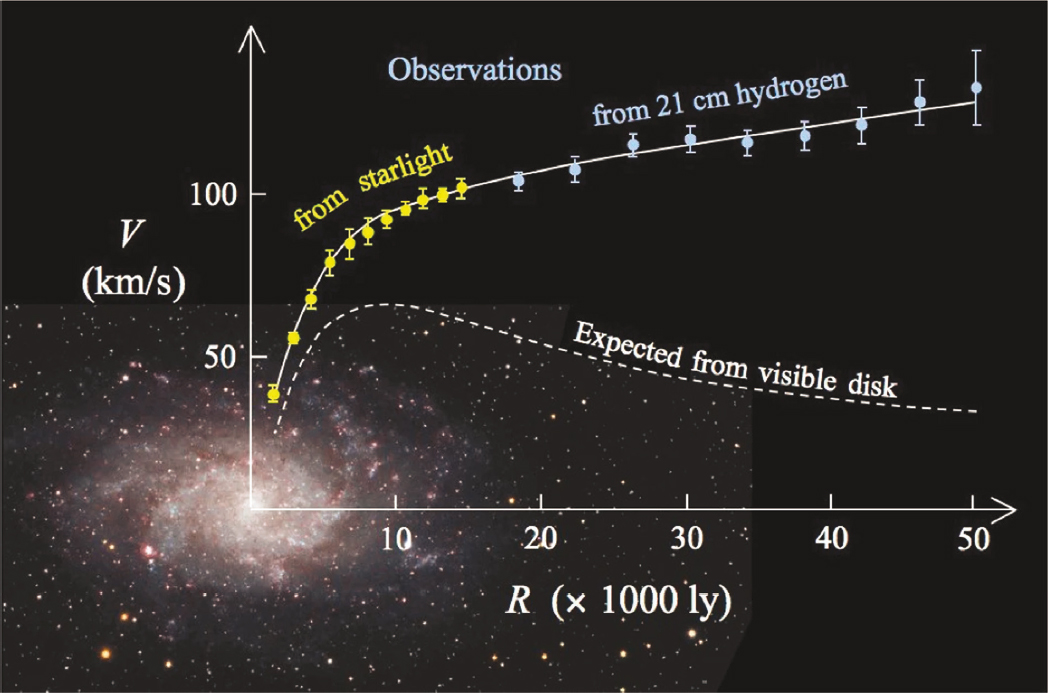
Are there violations of fundamental symmetries? Addressing any of these puzzles will be transformative even though the applications of such knowledge cannot be articulated today. This knowledge will change our current understanding of how the universe works and provide pathways to new frontiers. For example, solving the dark matter problem will not only reveal the composition of the universe but can also help us harness fundamental forces for technologies including quantum computing.
Indeed, while all of these questions seem to be purely fundamental, with minimal connection to practical applications and technology, it would be shortsighted to treat them as such. At the beginning of the 20th century, spectra of atoms, the photoelectric effect, and other puzzles of matter and radiation also seemed abstract and removed from human relevance. However, understanding these phenomena brought forth quantum mechanics, which became a cornerstone of modern technology. Atomic clocks enabling GPS navigation, electronics, lasers, nuclear magnetic resonance (NMR) spectroscopy, magnetic resonance imaging, superconducting magnets, electron microscopy, and much of modern chemistry are just a few examples. It is reasonable to expect that solving present fundamental physics problems—including via space-based experiments—will underpin future transformative technologies. Moreover, fundamental physics research in space extends beyond a quest for “new physics” and includes anticipated contributions to fundamental many-body quantum physics, predictions of phase transformations also embraced by the materials science and complex fluids communities, and methodological advances that may also advance adjacent fields such as astronomy and astrophysics.
As an example of the former, transformative advances in quantum technologies have led to a plethora of new high-precision quantum devices joining the search for dark matter, dark energy, and new forces; tests of
gravity; searches for violation of fundamental symmetries; sensitive measurements of gravitational waves; and many other investigations (Antypas et al. 2022; Safronova and Budker 2022; Safronova et al. 2018). In addition, the development of quantum devices has allowed direct tests of the underlying principles and implications of quantum mechanics and the study of the quantum versus gravity interface. Importantly, the accelerated development of high-precision quantum sensors largely happened after the 2011 decadal survey, and thereby reshaped the landscape of new physics searches over the past several years. The availability of these new techniques also gave rise to much theoretical work in particle physics, atomic, molecular, and optical (AMO) physics, gravitational physics, and other fields exploring new ideas. Together, these developments led to broad recognition of the discovery potential for space-based fundamental physics experiments. The enthusiastic response by the BPS research community to the request for input papers related to this decadal survey clearly demonstrates this strong interest, with a diverse array of opportunities identified for transformative fundamental physics investigations exploiting the unique space environment, away from Earth’s gravity. For example, some hypothesized dark energy and dark matter fields can be screened at the surface of Earth, reducing their measurable effects by many orders of magnitude. Furthermore, many fundamental physics experiments require a network of instruments placed at distances greater than Earth’s diameter—for example, long-wavelength/low-frequency detectors of gravitational waves. Other solar system objects (including the Sun, the Moon, and asteroids) could also provide access to unique fundamental physics explorations, including through direct studies of their behavior or as locations for precision measurement instruments.
A shared tenet of several open, fundamental physics sub-questions is that deploying quantum sensors and related precision measurement technology in space will enable transformative searches for new physics and/or major advances in our understanding of fundamental physics in ways not possible on Earth. However, such investigations are broad and open-ended by their nature. The rapid ongoing progress in the development of quantum sensors and precision measurement technology leads to the need for extensive effort to answer the first key physics question formulated above.
Several well-defined ideas and corresponding mission concepts have already been proposed (Alonso et al. 2022; Derevianko et al. 2022; Schkolnik et al. 2022). However, these are likely to barely scratch the surface of what space-based quantum and precision measurement experiments can offer to address the many outstanding questions of fundamental physics. For example, the idea of using atomic clocks to search for dark matter was first suggested only in 2014 (Derevianko and Pospelov 2014), with several other ideas generated since then, and research ongoing (Antypas et al. 2022). In addition to specific mission designs where the goals are already clear, a well-funded, multi-disciplinary, ground-based research program—including both experimental and theoretical investigations—is needed to form a healthy and vital interdisciplinary community of fundamental and applied physicists and experts in other fields to seek further ideas, hone techniques, and develop optimal mission plans and goals.
Note that companion infrastructure and technology investment will be important to the efficient success of addressing this KSQ. For example, deployment of reflector mirrors on Earth’s moon is an extremely low-risk/high-reward mission for improved tests of gravity and lunar conditions (Viswanathan et al. 2021).
Potential Research Areas
Search for New Physics with Clocks
As discussed in Chapter 2, the precision of space-based (optical atomic) clocks has improved by three orders of magnitude in the past 15 years, with expected additional orders of magnitude increased precision expected in the next decade (Brewer et al. 2019; Ludlow et al. 2015). Several such clocks, based on different types of atoms, have been demonstrated by multiple laboratories, with comparisons at the 10−18 level demonstrated over distance (Beloy et al. 2021).
Changes in gravity affect clock frequency, so comparing clocks in different gravitational potentials allows to test predictions of general relativity with exquisite precision as well as to map Earth’s gravitational potential (relativistic geodesy). Figure 5-6 summarizes the benefits of such research conducted in microgravity. In general relativity, gravity will affect all clocks in the same predictable way, but BSM (i.e., new) physics can change that, inducing clock shifts that depend on the type of the transition on which the clock is based, time, and space

location. Then, measurements of ratios of frequencies of identical clocks in different space (and gravitational potential) locations, measurement of ratios of frequencies for two different types of clocks (based on different transitions), and looking for either oscillatory or transient changes in such frequency ratios can discover a broad range of “new” physics (Antypas et al. 2022; Ludlow et al. 2015). The sensitivities of presently operating and future optical atomic clocks to new physics that affects the fundamental constants have been calculated from first principles to high accuracy. The effect of various types of dark matter and other new physics on clocks and specific search protocols have been established and tested on Earth where possible. Moreover, high-precision clocks have excellent long-term stability with negligible drifts, enabling autonomous navigation of spacecraft, including deep-space autonomous operation. As a result, atomic optical clocks in space have been proposed for a wide variety of fundamental and practical applications. Fundamental physics clock applications include tests of gravity, searches for dark matter and variation of fundamental constants, tests of Lorentz symmetry and local position invariance, measurements of gravitational waves (GWs) in unexplored parameter space (long-wavelength/low-frequency), and others.1 Practical applications include Earth-wide optical time and frequency metrology, ranging measurements, autonomous spacecraft navigation, relativistic geodesy, very long-baseline interferometry (VLBI), and others (Ely et al. 2021; Ludlow et al. 2015; Mehlstäubler et al. 2018).
Optical atomic clock operation outside the laboratory has been recently demonstrated (Ohmae et al. 2021), with several groups pursuing further work in this area. Automated operation, reducing clock size, weight, and power
___________________
1 See Alonso et al. (2022), Derevianko et al. (2022), Fedderke et al. (2022), Ludlow et al. (2015), Kolkowitz et al. (2016), and Schkolnik et al. (2022).
(SwaP) is ongoing as a part of the development of portable applications (Takamoto et al. 2022) as well as owing to sharing of related technologies and components with quantum information science and other applications. This concerted recent effort makes optical atomic clocks the most mature and versatile of the precision quantum sensors for space applications. Nonetheless, major technological development will be needed to transition and make ready optical atomic clocks for space use. An important point to stress is that the ability to compare different clocks and clocks in different locations is required to make use of the fantastic precision and stability of optical atomic clocks—that is, significant technological effort will also be required to implement optical time transfer between space- and ground-based optical atomic clocks.
Finding 5-4: Beyond optical atomic clocks, there is a diverse array of quantum and precision measurement technologies that will be critical to addressing space-based fundamental physics questions and associated applications, as outlined above. This topic has great potential to be scientifically transformative and is both enabling of space exploration and enabled by space.
Gravitational Effects on Quantum Optical Systems and Detectors
It is not clear whether gravity influences quantum entanglement. This potential influence is of fundamental interest in terms of quantum mechanical states, but with practical implications. Research that assesses this will require access to the space environment in LEO and lunar surfaces or pathways to Mars, with implications for Earth-based and longer-term quantum computing.
Many of the most promising space-based quantum and precision measurement technologies involve ultracold (laser-cooled) atoms and molecules (Alonso et al. 2022). Therefore, in addition to space-enabling experiments searching for new physics, it is important to study the behaviors of ultracold atoms and molecules in microgravity. The space environment will also enable the use of new trapping potentials to produce colder and denser atomic and molecular samples than possible on Earth, which will be enabling for many of the fundamental physics investigations described above. In addition, both atomic clocks and atom interferometry in space have been proposed for new types of long-wavelength/low-frequency GW detectors—key messengers of fundamental physical processes in the universe that are in the GW bands not accessible on Earth (because of seismic noise and Earth’s finite size) and outside the range of proposed space-based optical interferometer GW detectors such as LISA (Fedderke et al. 2022; Kolkowitz et al. 2016; Sedda et al. 2021). Therefore, extensive experiments to explore and understand cold atom and molecule behavior in microgravity are needed.
An intriguing possibility is the use of space-based quantum sensors as a part of multi-messenger astronomy—that is, looking for new physics signals correlated in time with GW detection (Dailey et al. 2021). In addition, atom-based GW space detectors can also serve as dark matter detectors (Abe et al. 2021; Antypas et al. 2022), so such instruments fall both within the “observation” and “fundamental physics” categories of space-based science.
Finding 5-5: In the search for new physics, fundamental physics and quantum science in space remain largely unexplored. In addition, quantum technologies are developing very rapidly, and the space program could adapt to benefit from new developments as quickly as possible. However, impactful progress and discovery requires synergistic and coordinated advances in ground-based and space mission infrastructure over more than one decade. That time horizon favors team-based science.
Recommendation 5-2: For fundamental physics in space, NASA should facilitate durable formation of collaborations and efficient knowledge transfer between researchers working in multi-disciplinary teams. This scope to address these multi-decadal key scientific questions should include ground-based infrastructure, theoretical and experimental physics, precision measurement and technology development with private sector participants, and should be coordinated with missions in which biological and physical sciences research is one among several whole-of-government objectives.
Impactful progress on the above four KSQs is enabled by predictable access to the space environment. In the coming decade as in past decades, access to space for BPS research will be a precious and limited resource. These KSQs represent the recommended focus of BPS resources and scientists based on current knowledge, not
because the answers will definitely advance specific space missions but rather because the space environment is key to advancing useful and transformative knowledge about how our world works. The current pace of change in knowledge on Earth—on these four KSQs spanning biophysics, physics, and properties of solids and complex fluids, and fundamental physics that requires and also offers measurement precision—has been impressive over the past decade. Thus, prudent planning and use of the space-based science and associated ground-based analogs can also anticipate surprise discoveries and innovations, prompting entirely new key questions and directions of research that are enabled specifically by access to the sub-orbital, orbital, and lunar surface environments in the coming decade. History indicates that emergent areas of space-enabled research are unlikely to be part of any existing roadmap for space exploration; the less we can predict what these future space-enabled areas are today, the more transformative they are likely to be for society in future decades. Likewise, multiple studies of which the space science and technology industry is aware (Hofstra et al. 2020; McSweeney 2021; Phillips 2014) indicate that teams with greater diversity of perspective and experience are favored to generate creative solutions to technical challenges.
Recommendation 5-3: In all of the space-enabled research areas, NASA should allocate funding with an anticipation that new directions of research may arise.
Chapter 6 outlines research campaigns as comprehensive, multi-year efforts that address audacious goals supporting space exploration and knowledge. These research campaigns are anticipated to provide partial informational or infrastructural support needed to address the KSQs outlined in Chapters 3–5, as well as those identified by the BPS community in the coming decade. (See Figure 5-7.)
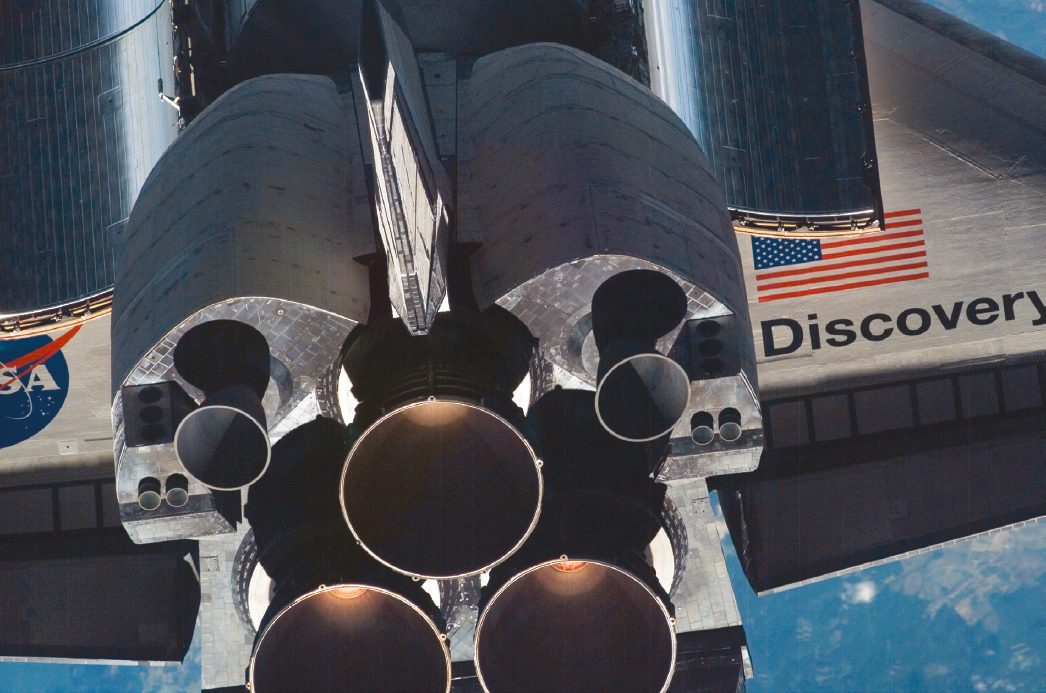