1
Introduction
An earthquake on the morning of February 9, 1971, produced a landslide in the upstream face of Lower San Fernando Dam (also known as the Lower Van Norman Dam), an earthen structure located at the terminus of the Los Angeles Aqueduct (see Figure 1.1). The dam crest dropped approximately 10 meters, leaving only about 1.5 meters of broken soil as freeboard to prevent overtopping and release of a reservoir holding approximately 15 million tons (approximately 13.6 million metric tons, or 4 billion gallons) of water (see Figure 1.1). Eighty thousand people were evacuated from the valley below. Many lives could have been lost had the water level in the reservoir been at maximum allowable pool level (Cortright, 1975) or the slide a bit larger (Page et al., undated). It would have been among the greatest earthquake-related disasters in U.S. history.
This near-disaster resulted from earthquake-induced liquefaction. Seismic shaking of sufficient strength and duration can transform certain saturated but firm soils1 into a suspension of soil particles and water that behaves in a manner similar to a viscous fluid. Under these conditions, the once-firm soil is transformed to one with low strength and stiffness. This phenomenon is called liquefaction (see Box 1.1), and in this report, the term “liquefaction” encompasses not only the conditions at the onset of phenomena (i.e., liquefaction triggering) but also the associated soil behaviors while in that state (e.g., flow liquefaction). At the Lower San Fernando Dam, the earthquake caused the soil within the dam to liquefy, and the loss of soil strength set off the landslide. The dam crest began to lower shortly after earthquake shaking began (Seed et al., 1975a; Castro et al., 1985; Gu et al., 1993). This example underscores the importance of assessing liquefaction hazards, both by determining whether a soil is likely to liquefy and by estimating consequences that may include, for example, damage to an earthen dam.
Accurate liquefaction assessment is essential to protect life and safety, to reduce seismic risk in a cost-effective manner, and to guide post-earthquake recovery. It is important for new construction and for enhancing the seismic safety of existing structures. However, liquefaction assessment can be challenging. The factors that govern liquefaction are often difficult to measure or predict, and the results of liquefaction analyses in engineering practice are sometimes contradictory: while one approach might indicate low liquefaction potential, another might warrant ground improvement costing millions of dollars. This report has its origins in such dilemmas that combine risk and uncertainty.
___________________
1 Soils, in this report, refer to particulate mineral Earth materials less consolidated than rock, as per geotechnical engineering practice. Soils contain readily visible particles (e.g., gravel and sand), particles visible only with magnification (e.g., silt and clay), or mixtures thereof.
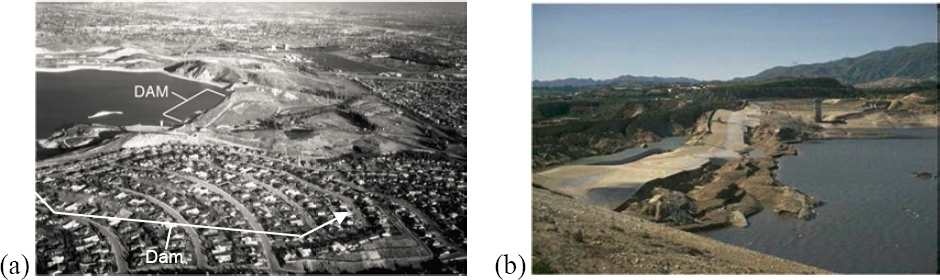
LIQUEFACTION HAZARDS
Large regions of the conterminous United States are susceptible to earthquake shaking strong enough to cause liquefaction in water-saturated soils. The shaded areas in Figure 1.2 highlight parts of the lower 48 states where the horizontal component of the peak ground acceleration2 (PGA) specified by the International Building Code (ICC, 2011) for residential and commercial construction is equal to or greater than 0.1 g (where g is the acceleration due to Earth’s gravity). In those shaded areas, liquefaction may be of concern wherever potentially liquefiable soils are present. (Note, however, depending on site-specific conditions, liquefaction cannot be precluded in the areas that are not shaded.) Historical and geologic evidence attest to the occurrence of liquefaction in about one-third of the lower 48 states. Table 1.1 provides representative examples of where liquefaction has occurred prior to 1964. These examples were identified through historical accounts or paleo-liquefaction investigations. The table includes cases in Arkansas, California, Illinois, Indiana, Kentucky, Louisiana, Massachusetts, Mississippi, Missouri, New Hampshire, Oregon, South Carolina, Tennessee, Virginia, and Washington (see Table 1.1).
The consequences of liquefaction include lateral and vertical ground displacements, landslides, slumping of embankments, foundation bearing failures, ejection of soil-water mixtures at the ground surface, buoyant uplift of buried structures, and increased lateral earth pressures on walls. These, in turn, can lead to settlement, distortion, and lateral displacement of the ground and the collapse of buildings; failure of earth-retaining structures; cracking, sliding, and overtopping of dams and highway embankments; rupture or severing of sewer, water, fuel, and other lifeline infrastructure; lateral displacement and shear failure of piles and pier walls supporting bridges and waterfront structures; and uplift of below-grade structures (see Figure 1.3 for examples). In an underwater setting, liquefaction-induced landslides can cause fast-arriving tsunamis on nearby shores and can be transformed into turbidity currents downslope that break submarine cables (Seed et al., 1988; Finn, 2003; Tinti et al., 2006).
___________________
2 Peak ground acceleration (PGA) is a most common measure of the intensity of ground shaking in a given area. The PGA is often used in engineering applications (e.g., liquefaction analyses, and in building codes).
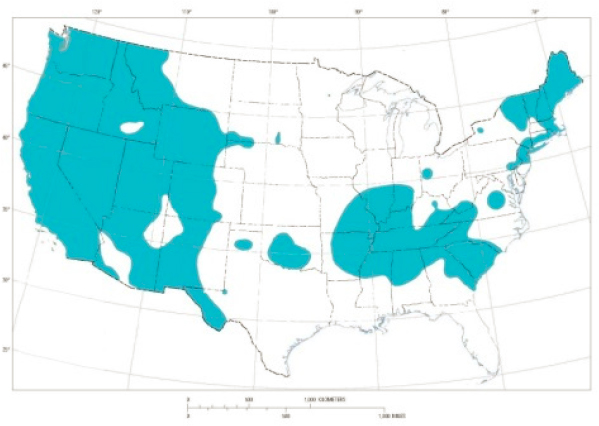
Liquefaction gained notice as an earthquake hazard in 1964 by causing major damage in Alaska and Japan. Liquefaction from the March 27, 1964 Prince William Sound region Alaska earthquake, of moment magnitude 3 (M) 9.2, caused widespread disruption to rail and road networks (McCulloch and Bonilla, 1970) and may have contributed to a landslide that generated a tsunami at the port of Valdez (Seed, 1968). Liquefaction from the June 16, 1964 Niigata earthquake in northern Japan, M 7.5, led to the damage of the Showa Bridge and the tilting of apartment buildings on reclaimed land adjacent to the Shinano River (see Figure 1.3). Earlier earthquakes, such as the M 9.5 Valdivia earthquake of May 22, 1960 in Chile, and the San Francisco, California earthquake of April 18, 1906 (M ~7.8), likely also resulted in liquefaction, but the phenomena of liquefaction had not yet been recognized at those times. Liquefaction was only identified in hindsight and therefore not extensively studied.
Damaging liquefaction has occurred during many subsequent earthquakes as well. In addition to the damage to the Lower San Fernando Dam described above, liquefaction induced by the M 6.6 San Fernando earthquake in 1971 caused lateral deformations of up to 1.5 meters on gently sloping ground over an area approximately 1,200 meters long and 270 meters wide (Youd, 1971; Holzer and Bennett, 2007) and damage to a water treatment plant then under construction for the California Water Project (Youd, 1973). The water treatment plant was to provide water for Ventura County, West Los Angeles, Santa Monica, and the Palos Verdes Peninsula. Liquefaction caused by the January 17, 1995 M 6.9 earthquake in Kobe, Japan (known as the Hyogo-ken Nanbu earthquake), destroyed almost all of the deepwater berths at what was, at the time, the 6th largest
___________________
3 The moment magnitude scale is the magnitude scale most commonly used by seismologists and earthquake engineers in current practice. Moment magnitudes provided throughout this report are those reported by the U.S. Geological Survey (http://earthquake.usgs.gov/earthquakes/eqinthenews) and may differ from values reported in the literature.
container port in the world. As a result, businesses and jobs went to ports in other countries, and, in spite of repairs, the port only recovered to be the 17th largest port in the world by 1997 (Chang, 2000) and the 28th largest by 2013.4 Liquefaction from the Christchurch, New Zealand, February 22, 2011, M 6.1 earthquake produced lateral spreading, settlement, and sand boils that devastated much of the city (see Box 1.2).
TABLE 1.1 Example Evidence for Pre-1964 Liquefaction in the Conterminous United States
State(s) | Evidence | References |
---|---|---|
Arkansas, Kentucky, Mississippi, Missouri, and Tennessee California | Sand boils from the 1811-1812 New Madrid earthquake and from prehistoric predecessors | Reviewed by Tuttle and Hartleb (2012)a |
Sand intrusions ascribed to prehistoric earthquakes of the past 1,800 years along the Hayward fault | Lienkaemper and Williams (2007) | |
Sand boils as much as 1,500 years old interpreted as evidence for earthquakes along the San Andreas fault north of Los Angeles | Sieh (1978) | |
Ground failures during the 1872 Owens Valley earthquake | Amos et al. (2013) | |
Ground failures during the 1906 San Francisco earthquake | Youd and Hoose (1978) | |
Ground failures during the 1933 Long Beach earthquake | Southern California Earthquake Centerb | |
Illinois and Indiana | Prehistoric sand dikes 1,500-7,500 years old in the Wabash Valley | Obermeier et al. (1991) |
Massachusetts and New Hampshire | Historical accounts of ground failures during the 1727 Newbury earthquake and sand dikes interpreted as evidence for preceding earthquakes in the past 4,000 years | Tuttle and Seeber (1991) |
Oregon and Washington | Sand intrusions along the Columbia River, ascribed to the 1700 Cascadia earthquake | Obermeier and Dickenson (2000); Takada and Atwater (2004) |
South Carolina | Ice-age lakebeds repeatedly deformed 17,000-23,000 years ago | Janecke et al. (2013) |
Sand boils from the 1886 Charleston earthquake and from preceding earthquakes of the past 6,000 years | Dutton (1889); Talwani and Schaeffer (2001) | |
Washington | Sand boils near Puget Sound, dated to about 1,100 years ago | Martin and Bourgeois (2012) |
Ground failures from the Puget Sound earthquakes of 1949 and 1965 | Chleborad and Schuster (1998) |
a This comprehensive report also reviews and catalogs evidence for liquefaction in the Wabash Valley and Newbury areas and additional parts of eastern North America: Charlevoix Seismic Zone of Quebec Province; Central Virginia Seismic Zone; Marianna, Arkansas; St. Louis, Missouri; and an area in southern Arkansas and northern Louisiana.
b See http://www.scec.org/education/080307longbeach.html.
___________________
4 See http://www.worldshipping.org/about-the-industry/global-trade/top-50-world-container-ports.
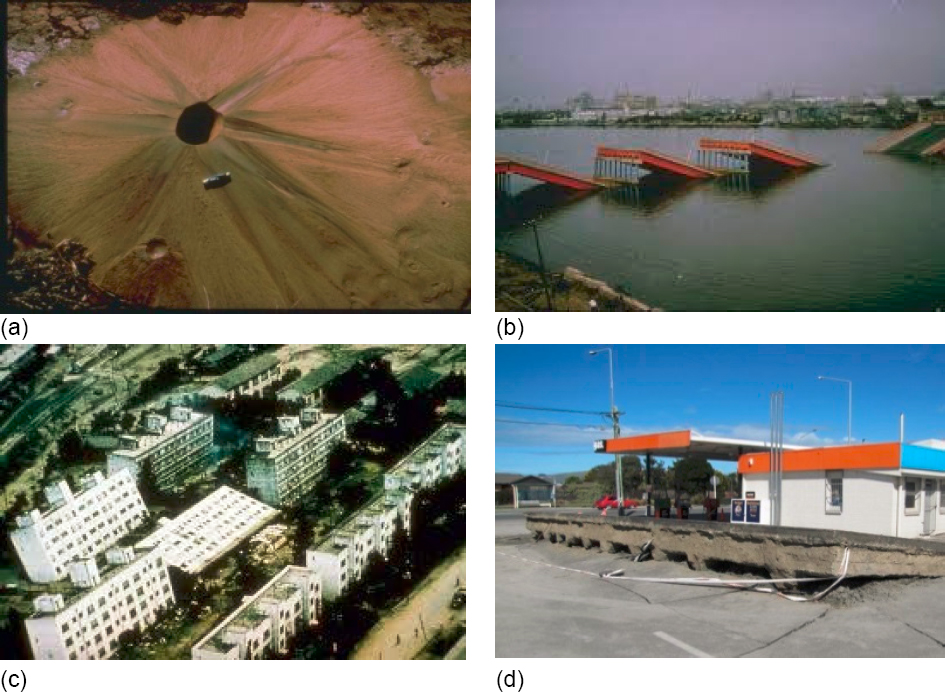
STATE OF PRACTICE FOR LIQUEFACTION ASSESSMENT
Across the United States, the state of practice for evaluating whether liquefaction can be expected and what its consequences may be varies greatly. Factors influencing the state of practice include the technical sophistication of the practitioners, their clients, and associated regulatory agencies; the size of a project and the severity of consequences given the occurrence of liquefaction; the type of project (e.g., dam, bridge, building, port and harbor facilities, or nuclear power plant); and associated technical literature, guidance documents, standards, and code provisions.
Higher levels of sophistication in liquefaction assessment, including site characterization and analyses of consequences, might be expected in areas where liquefaction is commonly recognized as a hazard, and among clients and regulators that work to mitigate the effects of liquefaction more frequently. Higher levels of sophistication may also be typical of liquefaction assessments for projects with major consequences given failure. Design guidance documents and documents that describe best practices for liquefaction assessment have been prepared for some specific classes of projects (e.g., for embankment dams [USBR, 2011], large dams [ICOLD, 2002], bridges [Dickenson, 2005; Kavazanjian et al., 2011, AASHTO, 2014], liquefied natural gas facilities [Bachman et al., 2007], ports and harbor facilities [ASCE, 1998], and nuclear power plants [USNRC, 2003]) as well as for general projects (CGS, 2008; SCDOT, 2010). Nevertheless, the level of detail provided in such documents varies widely. In some cases, design guidance may simply state that a liquefaction assessment should be conducted (OSBGE, 2014), while in other cases detailed requirements for site investigation and a specific step-by-step analysis procedure for liquefaction assessment may be provided (Kavazanjian et al., 2011).
Defining a standard of practice for liquefaction assessment is complicated by the rapid rate at which recommended approaches are developed and modified. For instance, a technical paper by Youd and colleagues (2001) describes techniques for assessment of the onset of liquefaction (also referred to as triggering) developed by consensus at community workshops sponsored by the National Science Foundation and the National Center for Earthquake Engineering Research at the State University of New York at Buffalo. By 2004, as a result of continued investigations on the topic, alternative sets of changes to the consensus procedures were suggested by reputable groups, with much emphasis placed by the technical community on two alternate approaches (Boulanger and Idriss, 2004a; Cetin et al., 2004). Since then, liquefaction assessment in current engineering
practice has been inconsistent. Awareness of (and concern about) those inconsistencies has been demonstrated in the results of a 2012 survey conducted by the Deep Foundations Institute of engineers and contractors from the public, private, and academic sectors (Siegel, 2013).
PRIOR REVIEWS OF LIQUEFACTION ASSESSMENT
Simplified approaches to estimate whether liquefaction is likely to be initiated (i.e., to determine if the stiffness and strength of the soil may drop significantly due to liquefaction) were proposed by Whitman (1971) and Seed and Idriss (1971). Most engineers have since relied on variants of the Seed and Idriss (1971) basic approach (see Box 1.3). In 1985, the National Research Council (NRC) held a workshop on liquefaction and issued a report that summarized advances in liquefaction triggering assessments since the Alaska and Niigata earthquakes (NRC, 1985). In 1996 and 1998, the National Center for Earthquake Engineering Research (NCEER) held two workshops on earthquake-induced soil liquefaction. The report issued following those workshops (Youd et al., 2001) represents the most recent point at which a general consensus on liquefaction triggering procedures was achieved. It is important to note that those workshops and reports addressed liquefaction triggering only; they did not address issues associated with liquefaction consequences.
Several groups have suggested alternatives to some of the NCEER workshop recommendations (e.g., Zhang et al., 2002; Cetin et al., 2004; Bray and Sancio, 2006; Moss et al., 2006; and Idriss and Boulanger, 2008). There can be substantial differences among these revisions—relative to each other and relative to those in the NSF/NCEER workshop publications (Youd and Idriss, 1997; Youd et al., 2001). The differences can be large, especially when evaluating liquefaction triggering at depths greater than approximately 15 to 18 meters below grade and in soils containing significant amounts of silt and clay particles (i.e., greater than 5% by weight). Views differ on which revisions are most appropriate for liquefaction triggering assessment. Further, as noted by Youd and colleagues (2001), there has never been consensus on such issues as how best to assess liquefaction triggering on non-level ground (i.e., for slopes greater than 6% or for embankments and dams) or on liquefaction consequence assessments.
Several alternatives to the simplified stress-based approach to assess liquefaction triggering have been suggested. Strain-based approaches (Dobry et al., 1982; Dobry and Abdoun, 2011) and energy-based approaches (Davis and Berrill, 1982; Berrill and Davis, 1985; Law et al., 1990; Kayen and Mitchell, 1997; Green, 2001; Kokusho, 2013) have been proposed but are not used widely in practice. Furthermore, liquefaction assessment has expanded beyond that of triggering. It is no longer sufficient to assess whether liquefaction will occur at a site; it is necessary to evaluate its potential effects on the strength, stability, and deformation of the ground and the consequences on nearby structures and facilities. Various approaches to evaluate the consequences of liquefaction have been developed over the past two decades (Ishihara and Yoshimine, 1992; Martin et al., 2002; Youd et al., 2002; Ishihara and Cubrinovski, 2004; Kavazanjian et al., 2011). While development of analysis methods for liquefaction consequences is a positive step, considerable uncertainty remains in the prediction of liquefaction triggering and its consequences.
THE COMMITTEE’S TASK
In 2010, an ad hoc committee of the Earthquake Engineering Research Institute (EERI) called for a community-based evaluation of the state of the art in liquefaction assessment (Finn et al., 2010). The EERI committee recommended that the assessment be conducted under the auspices of the National Academies of Sciences, Engineering, and Medicine (the National Academies) to help build consensus within the community and to lead to more confidence in methods used to assess liquefaction potential and consequences. This report is the culmination of the efforts in response to that recommendation from the EERI. Under the sponsorship of the American Society of Civil Engineers, an award from the Los Angeles Department of Water and Power, an award from the Port of Long Beach, an award from the Port of Los Angeles, Grant No. R11AP81544 from the U.S. Bureau of Reclamation, Award No. DTFH6114P00075 from the U.S. Department of Transportation’s Federal Highway Administration, and Award No. NRC-HQ-12-G-04-0061 from the U.S. Nuclear Regulatory Commission, the National Academies convened a committee of 12 engineers and scientists to solicit input from the technical community and to critically examine the state of practice in liquefaction hazard and consequence assessment. The complete Statement of Task as agreed to by the National Academies and the study sponsors is provided in Box 1.4.
The Statement of Task requires the committee to “assess and evaluate” various methods and assessment approaches. The committee considered the difference between “assess” and “evaluate” and determined the terms to be synonymous in this context. They are used interchangeably throughout this report. Although the task requires the study committee to evaluate objectively the various assessment methods (i.e., specific assessment procedures or techniques), the task does not ask the committee to adjudicate disputes regarding any specific methods or to recommend new best practices; it is not the intent of the committee to do so. The committee has sought to identify and constrain, where possible, uncertainties in various assessment methods and to identify what could be done in the near and long terms to advance research and practice. Because many assessment approaches applied today are often modifications of past approaches, and because much if not most of the data employed in more contemporary approaches were collected prior to 1996, the committee both describes early methods and databases to determine the uncertainties associated with them and also looks at contemporary assessment approaches to determine how those uncertainties may have been mitigated, exacerbated, or compounded. The report addresses evaluation of earthquake-induced liquefaction only of naturally occurring soils and man-made fills composed of such soils. The study scope does not extend to mine tailings, solid waste, or stabilized soils. Assessment of remedial measures for increasing liquefaction resistance and mitigation of post-liquefaction consequences is also outside the scope.
Committee Member Selection
Committee members were nominated by their peers and selected by the National Academies from the resulting long list of nominees based on the strength of their professional qualifications using long-standing procedures established by the National Academies to adhere to the highest standards of impartiality and independence. The committee includes researchers and practitioners with expertise in soil properties and behavior, in situ and laboratory testing, ground motion propagation and attenuation, quantitative hazard and risk assessment, seismic site response analysis, geological evidence of liquefaction, engineering geology, geotechnical earthquake
engineering, soil-structure interaction, and computational mechanics. Brief biographies of the committee members are given in Appendix A.
Information Gathering
Technical input from the engineering community was vital to the study process. Committee conclusions and recommendations in this report are based on synthesis and assessment by the committee of publicly available information and data. Additional information provided to the committee by researchers during the conduct of this study was given with the understanding that all information was subject to public disclosure. No primary research was conducted by the committee. This report reflects information available to the committee as of early 2015. Information made available later—for example, during the November 2015, 6th International Conference on Earthquake Geotechnical Engineering in New Zealand—would have been available too late for the study committee to deliberate its significance to this report and its recommendations.
The committee organized and held a 2-day public workshop with approximately 90 participants and held three additional public meetings and a webinar devoted to various topics. Meetings were announced widely in advance through several communication mechanisms; attendance at the workshop and public meetings was open to all interested parties; and remote participation was possible for all of these sessions. Agendas for these meetings are provided in Appendix B, as is a list of workshop participants. Solicitations for written input from any member of the community on any relevant topic were made during all open session meetings. The committee also requested written clarification on specific issues from individual members of the technical community.
REPORT ORGANIZATION
Engineering practice related to earthquake-induced soil liquefaction is informed largely by empirically based methodologies that are themselves informed by somewhat disparate perspectives and uneven availability of data. Understanding the uncertainties associated with various approaches and data is similarly uneven. Uncertainty, then, is an overriding theme in this report because it is through an understanding of uncertainty that sources of inaccuracy are identified. Consideration of uncertainties becomes even more important as earthquake engineering practice more generally moves toward performance-based analysis and design.5
This report is organized as follows:
- Chapter 2 provides a description of the phenomena associated with earthquake-induced soil liquefaction and the factors influencing them;
- Chapter 3 discusses the sufficiency of the case history data on liquefaction and associated phenomena, including field case histories, and provides a critical assessment of those data;
- Chapter 4 describes and assesses the simplified stress-based approach to predict the triggering (initiation) of liquefaction;
- Chapter 5 assesses alternative approaches to liquefaction triggering assessment such as strain-based, energy-based, and computational mechanics-based approaches;
- Chapter 6 describes the assessment of the post-liquefaction shear strength of soils;
- Chapter 7 discusses empirical and semiempirical methods to evaluate liquefaction consequences;
- Chapter 8 is a discussion of how computational mechanics can be used to predict liquefaction triggering and consequences;
- Chapter 9 is a discussion of performance-based engineering methods for probabilistic evaluation of liquefaction susceptibility, triggering, and consequences; and
- Chapter 10 provides a synthesis of committee recommendations for advancing the state of practice and state of the art for assessment of earthquake-induced soil liquefaction.
___________________
5 Performance-based design is a process in which the performance of a facility being designed is evaluated over the entire range of possible loadings, rather than for one or more discrete ground motion return periods or events. The approach is intended to allow rational decisions regarding appropriate design levels, including decisions related to the added value of increasing the seismic resistance. Financial, construction, and operational issues are considered over the life cycle of the facility. The approach usually involves probabilistic descriptions of earthquake ground motions and the facility’s response to them.
Summaries of the key findings and conclusions derived from each chapter can be found in a box at the beginning of the chapter, beginning with Chapter 2. A more detailed explanation of each of the bulleted items in the key findings boxes is found within the text of the chapter. These key findings and conclusions also inform the committee’s major recommendations, found in Chapter 10.