4
Radiation Sources and Alternative Technologies in Medicine and Research
This chapter discusses some major applications of radioactive sources in medicine and research and the current status of alternative technologies for replacing them. References to specific technologies and in some cases to specific commercial products and manufacturers do not necessarily constitute or imply their endorsement by the committee.
4.1 BLOOD IRRADIATION
Blood irradiation is the most common method used in the United States to prevent transfusion-associated graft-versus-host disease (TA-GvHD). TA-GvHD occurs when a cellular blood component containing T cells from an immunocompetent donor is transfused to an immune-incompetent recipient (host). The transfused donor cells react against the recipient’s tissue and cause damage to the skin, liver, and gastrointestinal tract. Most importantly, in TA-GvHD, the donor immunocompetent leukocytes also attack the host’s bone marrow and produce a fatal hypoplastic or aplastic bone marrow failure state. The host often dies of infection due to sepsis. TA-GvHD also occurs when the donor and recipient human leucocyte antigen type is similar as can occur in blood donations from first-degree relatives. In these cases, the recipient’s immune system cannot identify the donor lymphocytes as foreign and destroy them (Bahar and Tormey, 2018). As there are few, if any, effective therapeutic interventions once TA-GvHD occurs, the only approach is to prevent it from occurring in the first place.
Reducing the risk of TA-GvHD is based on blocking T-cell replication by treating cellular blood products prior to transfusion into a susceptible patient. In the United States and Europe, only blood components used for the treatment of patients with identified risk require treatment such as irradiation. Approximately 16 percent of red cell and 52 percent of platelet units in the United States were prophylactically irradiated with gamma or x-ray sources in 2017 to prevent TA-GvHD in susceptible recipients (Sapiano et al., 2020). Percentages of treated blood components vary significantly by country based on risk for TA-GvHD. Risk is higher in genetically more homogeneous populations, such as in Japan, as compared to populations with greater degrees of heterogeneity, such as those in many African countries.
The following sections discuss the capabilities of different technologies for prophylaxis against TA-GvHD. These are also summarized in Table 4.1.
TABLE 4.1 Capabilities of the Different Technologies with Respect to Prophylaxis Against TA-GvHD and Pathogen Reduction
Isotopic Technology | Nonisotopic Technology | ||||
---|---|---|---|---|---|
Gamma (Cs-137) Irradiator | X-ray | UVA Pathogen Reduction (Amotosalen) | UVB Pathogen Reduction (Riboflavin) | UVC Pathogen Reduction | |
Whole blood | Yes | Yes | No | (Yes) | No |
Platelets | Yes | Yes | Yes | (Yes) | (Yes) |
Plasma | Yes | Yes | Yes | (Yes) | No |
Red blood cells | Yes | Yes | No | (Yes) | No |
Transfusion-associated graft-versus-host disease (T-cell inactivation) | Yes | Yes | Yes | (Yes) | (Yes) |
Transfusion-transmitted infections | No | No | Yes | (Yes) | (Yes) |
Transfusion-associated adverse reactions | No | No | Yes | (Yes) | (Yes) |
NOTES: (Yes) means not available in the United States. TA-GvHD = transfusion-associated graft-versus-host disease; UVA = ultraviolet A; UVB = ultraviolet B.
SOURCE: Adopted from NSTC, 2016, and updated.
4.1.1 Radioisotope Technologies
Gamma irradiation of blood components with doses that abrogate the ability of white blood cells (WBCs) to proliferate is the predominant method to prevent TA-GvHD. Gamma irradiation of blood components to inactivate lymphocytes is the predominant method to prevent TA-GvHD (see Figure 4.1a). Dedicated cesium-137 blood irradiators (incorporating pressed cesium chloride (CsCl) powder sealed in stainless steel capsules; referred to as cesium irradiators in this chapter) have been the standard method for irradiation of blood components to inactivate lymphocytes and prevent TA-GvHD for decades. Blood irradiation is the most common application using cesium-137, followed by research irradiators (see Section 4.2). Cesium irradiators are a Category 1 source based on the International Atomic Energy Agency’s (IAEA’s) categorization system and typically contain multiple sources totaling 1,000–5,000 Ci (37–185 TBq) of CsCl at the time of purchase. About 5 percent of blood irradiators use high-activity cobalt-60 (NRC, 2008). The radioactive source is completely contained in lead shields, so blood irradiators are called “self-shielded” irradiators. Including their shields, these irradiators are very heavy, weighing more than a metric ton. The National Nuclear Security Administration (NNSA) estimates that there are approximately 400 cesium blood irradiators in the United States (Itamura et al., 2018).
For TA-GvHD prophylaxis, current guidance from the Food and Drug Administration (FDA) recommends that 25 Gray (Gy) must be directed to the midplane of the irradiation canister, with no dose within the canister less than 15 Gy (FDA, 1993). Cesium irradiators are designed so that the operator is automatically protected by shields as the canister holding the blood products is moved into the irradiation chamber. The irradiation canister is rotated on a turntable for dose uniformity. Doses do not typically vary within the canister by more than 20 percent, except at a small portion in the top and bottom of the canister (NRC, 2008). Because of natural radioactive decay, cesium and cobalt irradiators are dose-mapped annually and exposure times are recalculated (Moroff and Luban, 1997) to ensure that the FDA-recommended dose is applied to blood components. Unless the blood irradiator is nearing, or is beyond, the end of its life (older than about 30 years) exposure times are typically about 5 minutes. Today’s cost of purchasing a cesium irradiator ranges from $200,000 to $350,000.
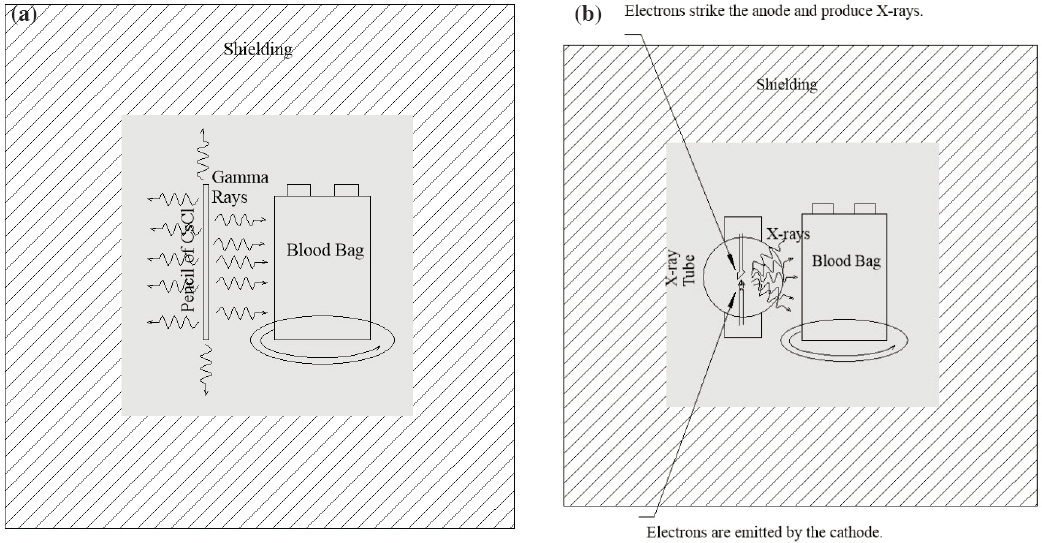
4.1.2 Alternative Technologies
There are two alternatives to cesium-irradiators: x-ray irradiators for all blood components and pathogen reduction methods for some blood components. These are discussed in the following sections.
X-ray Irradiators
X-ray blood irradiators were first approved by FDA as an alternative to cesium irradiators in 1999. During the early years of implementation, x-ray irradiators were prone to frequent breakdowns as compared to gamma irradiators due to x-ray tube or power source failures. Several blood banks purchased backup x-ray irradiators or entered into cooperative agreements with nearby institutions to compensate for breakdowns (NRC, 2008).
In February 2009, FDA granted approval for the RS3400 x-ray blood irradiator from Rad Source Technologies that incorporated a redesigned x-ray tube (FDA, 2021b). The new tube was elongated and held a linear array of cathode filaments surrounded by an x-ray transmissible coaxial target anode made of gold (Au), which is a target material with a higher atomic number. The larger surface area of the anode as compared to traditional x-ray tubes allowed better heat dissipation. With multiple short cathode filaments and the higher-atomic-number target, it produced greater radiation output 360 degrees around the filament with lower voltage, lengthening the life of the x-ray tube (Ausburn, 2016). Samples are rotated coaxially around the single tube to provide homogeneous irradiation. Tubes with damaged targets can be refurbished by the manufacturer rather than being disposed of. Because of the lower voltage requirement and better heat dissipation, the device containing the redesigned x-ray tube (referred to as second-generation x-ray tube in this report) can be cooled using a closed water circulation system rather than having to be connected to public water, a requirement of the first-generation x-ray devices. The second-generation x-ray blood irradiator technology deployed within the past 10 years has been broadly accepted as a reliable and cost-effective alternative to cesium irradiators. Figure 4.1b is a schematic representation of an x-ray blood irradiator.
Currently, at least four manufacturers produce or distribute x-ray irradiators: Rad Source Technologies produces the RS3400 irradiator; Hitachi produces the Sangrey irradiator; R3 X-Ray LLC distributes RadGil2 US; and
Best Theratronics produces the Raycell MK2. Of these products, the RS3400 contains the second-generation tube described earlier in this section and the other products contain first-generation tubes. The device configurations vary, but first-generation x-ray irradiators typically use a conventional x-ray tube system of two tubes enclosed in a lead-shielded container. To ensure a uniform radiation dose without target rotation, the device may use two opposing x-ray tubes, one on each side of the chamber where the target container is placed, and dose delivery is controlled by setting and monitoring the irradiation time, based on the central dose rate.
The throughput of x-ray devices is comparable to cesium irradiators with typical exposure times of about 5 minutes. The x-ray devices are about the same size as gamma irradiators but weigh slightly less. The cost to purchase an x-ray blood irradiator is comparable to a cesium irradiator, about $270,000 (NTI, 2018a).
Stellarray, a small business recipient of NNSA’s Small Business Innovation Research (SBIR) and Small Business Technology Transfer awards is developing a range of irradiators for isotope replacement based on a new x-ray source architecture called the flat-panel x-ray source (FPXS) including a self-contained blood irradiator (SCBI). Unlike conventional x-ray systems in which x-rays are generated from a small spot on an angled or curved anode, FPXS produces x-rays over a much broader area on a flat anode surface. The exposure of one side of the anode to the external environment for cooling, the absence of the heel effect of an x-ray tube, and the ability to make the source flux area the same as the radiation target area provide advantages in terms of power efficiency and thermal management. Figure 4.2 shows a cross-sectional view of an FPXS panel. The technology configuration is described in some detail elsewhere (Eaton et al., 2019).
Design of the FPXS started more than 10 years ago when issues of reliability of x-ray sources for blood irradiation were prohibitive for their use in this application. The SCBI is designed to deliver a uniform dose of 25 Gy to blood bags and other blood products and to achieve no more than 10 percent variation in dose along any axis, which is what is typically achieved with x-ray machines currently in the market. One possible advantage of this system is that it can irradiate blood in less than 4 minutes, making it well suited for use in busy blood banks and hospitals. Also, the cabinets for these irradiators are about one-fourth the size of currently available irradiators using either x-rays or gamma rays. Except for these possible advantages, Stellarray’s technology does not appear
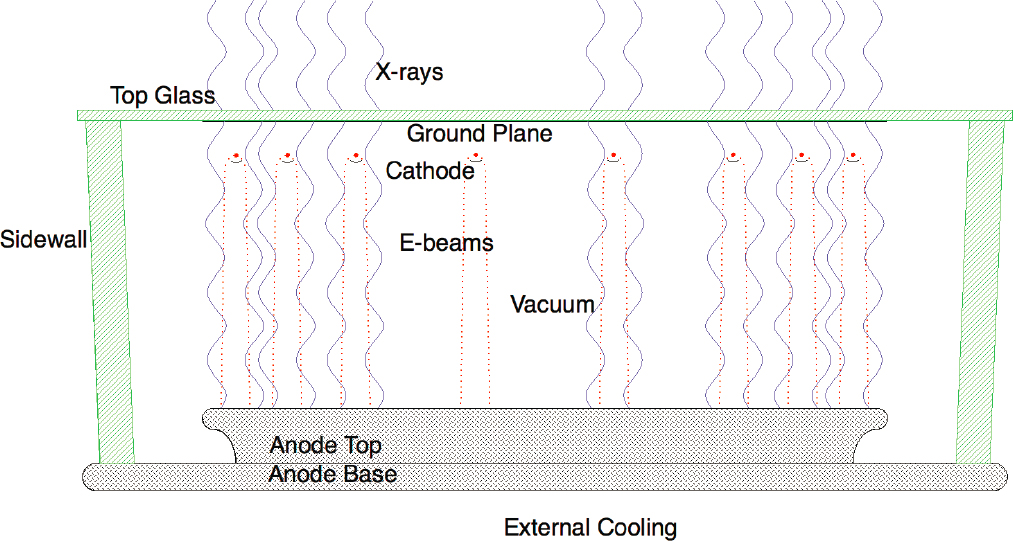
SOURCE: Courtesy of Stellarray, Inc.
to provide an obvious benefit to x-ray systems for blood irradiation already in the market, unless it is priced attractively.1 However, this new technology, which is expected to come into market in 2021, will add competition in the product market. Two types of research irradiators and an insect sterilization irradiator are also under development using the same basic system architecture.
The feasibility of using medical linear accelerators (linacs) to irradiate blood components has been demonstrated in a number of studies (Butson et al., 2000; Olivo et al., 2015; Pinnarò et al., 2011), but exploring this option only makes sense in settings where there is no option for a dedicated blood irradiator due to limited resources. The challenge in these studies has been primarily establishing the dosimetry plan to achieve a homogeneous dose distribution. Several companies have attempted to develop the next generation of low-energy compact accelerators dedicated to replacing radioisotopes in cesium blood irradiators. For example, in 2010, RadiaBeam Systems developed a novel fabrication process for linacs with the goal to reduce the costs and size, making them suitable for these applications. The accelerator, which they termed the MicroLinac, was developed at the Stanford Linear Accelerator Center, a Department of Energy (DOE) facility. It was powered by a commercially available x-band magnetron radio frequency (RF) source. A 2-MeV linac prototype for self-contained irradiators was built and tested but was deemed too expensive for application.2 Since 2016, RadiaBeam has been pursuing the approach of split linac fabrication, capable of significant reduction in fabrication costs due to fewer components. RadiaBeam’s developments in this area range from 0.2- to 1.0-MeV accelerators for portable radiography to more powerful 6-MeV systems for irradiation applications.
Pathogen Reduction Methods
Pathogen reduction (or pathogen inactivation) is a process whereby blood components are treated soon after collection to inactivate any remaining infectious agents including viruses, bacteria known to contaminate platelets,3 and parasites. Aside from that contained in leukocytes (including T cells), there should not be any genomic nucleic acids (DNA or RNA) in human blood for transfusion. Any such DNA or RNA present in collected blood is that associated with a pathogen. Pathogen reduction is achieved using methods that modify the nucleic acids of the pathogens in the different blood components.4 The need to manufacture pathogen-reduced blood components differs by component. For example, the need to manufacture pathogen-reduced platelets is higher than that of manufacturing pathogen-reduced plasma. This is because platelets are stored at room temperature, and therefore there is heightened risk of bacterial growth if the component unit is subject to contamination. Plasma is stored frozen, and bacterial contamination is less likely to occur in a cryopreserved component.
In the context of this report, treatment of some blood components with pathogen reduction methodologies has been found to be effective against proliferation of lymphocytes and therefore in preventing TA-GvHD. This makes pathogen reduction methods alternatives or possible alternatives to cesium and cobalt-60 irradiators for the treatment of some (but not all) blood components. Although some pathogen reduction methodologies can be alternatives to gamma radiation, gamma radiation has been reported as an ineffective alternative to pathogen reduction (Bello-López et al., 2016).
FDA approved two ultraviolet (UV) systems from one manufacturer for treatment of platelets and plasma to achieve blood pathogen reduction in 2014. Systems for pathogen reduction of red blood cells or whole blood treatments have not yet obtained FDA clearance.
The first pathogen reduction method for platelets and plasma involving a psoralen, amotosalen, and ultraviolet A (UVA) light was licensed to Cerus Corporation (FDA, 2014) and has been in use in some countries in Europe since the early 2000s. The treatment inactivates viruses, bacteria, parasites, and lymphocytes by producing amotosalen adducts in nucleic acid, blocking pathogen and lymphocyte replication with retention of blood component therapeutic function (Castro et al., 2018; Grass et al., 1998) (see Figure 4.3). The density of adducts in lymphocyte
___________________
1 The target price is $175,000 to $275,000 according to CISA (2019).
2 Salime Boucher, RadiaBeam Technologies, LLC, presentation to the committee on December 17, 2020.
3 FDA updated its guidelines for bacterial risk control strategies in December 2020. The new guidelines require additional safety measures, which will likely lead to increased utilization of pathogen reduction methods in the United States (FDA, 2020).
4 Some damage has been observed in the quality of red cells and platelets subjected to pathogen reduction, but decrements have been judged acceptable based on approvals by regulatory bodies.
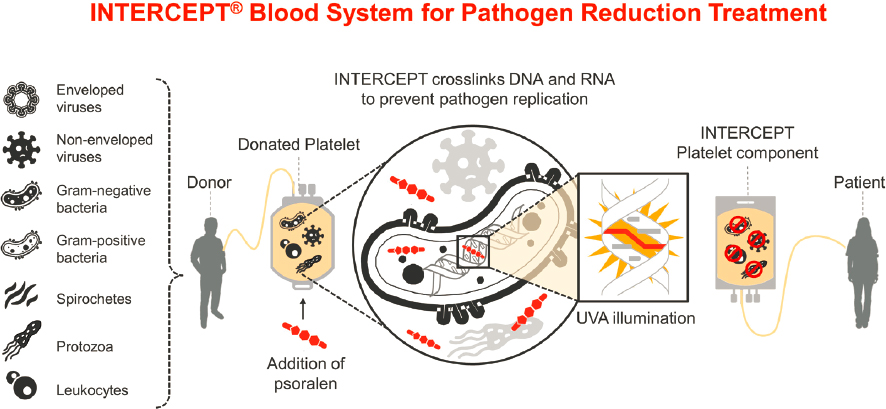
SOURCE: Cerus Corporation.
DNA produced by amotosalen and UVA (approximately 1 in 37 base pairs) is significantly greater than the density of DNA breaks caused by 25 Gy of gamma or x-ray irradiation (1 in 37,000 base pairs), suggesting greater replication inhibition and hence greater inactivation of lymphocytes (Castro et al., 2018).
In platelets, amotosalen and UVA treatment was recognized as prophylactic to reduce the risk of TA-GvHD and became part of the AABB (formerly the American Association of Blood Banks) standards in 2016 (Regan and Markowitz, 2016) and is currently practiced at many blood centers. As of April 2021, approximately 50 percent of the American Red Cross apheresis platelet collections were treated with this technology with a target of 100 percent pathogen reduction of apheresis platelet collections with this technology by 2023.
Cerus has a similar system for pathogen inactivation of plasma (FDA, 2021a) and for the manufacture of pathogen-reduced cryoprecipitated fibrinogen complex derived from plasma (FDA, 2020), which is licensed for the treatment and control of massive hemorrhage associated with fibrinogen deficiency. T-cell leukocyte contamination of frozen plasma is infrequently considered a risk factor for TA-GvHD, and gamma irradiation is rarely used; however, some T cells can remain viable after freezing. Liquid plasma never frozen is transfused to some patients and rarely treated with gamma irradiation. The amotosalen-UVA technology for plasma received an FDA intended use indication to reduce the risk of transfusion-transmitted infection and as an alternative to gamma irradiation for prevention of TA-GvHD for frozen and liquid plasma. However, although FDA-approved, plasma pathogen-reduced by this technology is not available in the United States, partly because of manufacturing difficulties and partly because of resource constraints at blood centers.
The second pathogen reduction system for platelets utilizes riboflavin and UVB light. The method was in Phase 3 clinical trials in the United States (NLM, 2017) until the trial ended because it could not meet primary study endpoints. However, this method has been an approved method for use in Europe since the early 2000s. The treatment causes nucleic acid base modification and strand cleavage (Mundt et al., 2014) in pathogens and inactivates lymphocytes (Fast et al., 2011). Riboflavin and UVB have also been investigated in pathogen inactivation for whole blood in pre-clinical studies and clinical trials (Yonemura et al., 2017). Whole-blood pathogen reduction can potentially produce both platelet and red cell inactivated blood components by standard blood bank centrifugation methods.
UVC light alone (without a photosensitizing agent) has been successfully investigated for pathogen reduction and the inactivation of white blood cells in platelet components, including the prevention of TA-GvHD in an animal model (Pohler et al., 2015; Seltsam and Muller, 2011). The UVC method, named the THERAFLEX UV--
Platelets system (MacoPharma, Mouvaux, France), is still undergoing clinical development and is not currently licensed in any market.
As noted previously, systems for pathogen reduction of red blood cells have not yet obtained FDA clearance. UV light methods are more difficult to apply to red cell inactivation because hemoglobin A present in red blood cells absorbs UVA and dampens the crosslinking that occurs in psoralen-treated red cells. Cerus has been investigating the use of a nucleic acid–targeted alkylating acridine–derived labile alkylating agent, amustaline (S-303), for the inactivation of viruses, bacteria, parasites, and lymphocytes in red blood cells for the past decade (Henschler et al., 2011; North et al., 2011). Amustaline red blood cell products have been studied in Phase 2 clinical trials in the United States (Cancelas et al., 2017) and in two Phase 3 clinical trials in Europe (Aydinok et al., 2019; Brixner et al., 2018). The European CE Mark registration is in review. Amustaline red blood cells are currently in two Phase 3 trials in the United States. Results from these trials could be expected within the next 3 to 4 years.
4.1.3 Alternative Technology Adoption Considerations
Broad adoption of x-ray irradiators for blood irradiation occurred following the implementation of the Cesium Irradiator Replacement Project (CIRP) (see Sidebar 1.2), and this broad adoption continues to occur. CIRP provides financial incentives toward the purchase price of a new nonradioisotopic device, as well as the removal and disposal of the cesium irradiator. To date, CIRP has helped remove 165 cesium irradiators, and another 150 irradiators are being scheduled for removal. Outside of CIRP, there is limited tracking at the national level of adoption rates of alternative technologies for cesium irradiators. A survey indicated that about 75 percent of the blood components irradiated in 2017 were done so using cesium irradiators and the remainder with x-ray technologies (Sapiano et al., 2020).
X-ray blood irradiators are recognized as a suitable and economic alternative to gamma irradiation. Bakken and colleagues (2013) performed a cost-benefit analysis comparing cesium and x-ray blood irradiators. Cost comparisons were performed at three levels of utilization of the irradiator depending on the annual number of blood units irradiated. These comparisons considered costs incurred from securing and disposing of cesium sources, operational and maintenance costs, as well as societal costs. The study concluded that in most cases, there is a cost benefit to switching to x-ray technologies for this application (Bakken et al., 2013).
A representative from Mount Sinai Hospital in New York City, a leading institution in the adoption of x-ray technology to replace cesium blood and research irradiators, presented to the committee the institution’s cost-benefit analysis, which also demonstrated financial savings by transitioning to x-ray irradiators. As of January 2018, Mount Sinai migrated completely to x-ray irradiators and disposed of its cesium irradiators through CIRP (Kamen et al., 2019). The effort took 9 years to complete. A similar cost-benefit analysis conducted by a smaller medical center, the Christus Spohn Hospital in Texas, also demonstrated financial savings by transitioning to the x-ray irradiator.5
The American Red Cross, the largest blood treatment and supply organization in the United States, began replacing its cesium irradiators with x-ray irradiators in 2017. At that time, the American Red Cross had 33 blood irradiators. As of April 2021, 27 cesium irradiators have been removed. The organization has installed a total of 37 x-ray irradiators at 34 sites to replace cesium irradiators and aging x-ray irradiators. Very few major repairs of the x-ray devices have been required despite heavy daily use.
Broad adoption of x-ray technology for blood irradiation has also taken place elsewhere. Japan started replacing cesium blood irradiators 20 years ago, and 80 percent of them have been replaced by x-ray irradiators. According to a report, the burden of regulations and the fear of radioactivity by the Japanese public in the aftermath of the Fukushima Daiichi nuclear power plant accident were factors that accelerated the use of x-rays to irradiate blood (Bieniawski et al., 2017). In Norway, the July 2011 terrorist attacks targeting government and public infrastructures were the impetus for Norwegian authorities to enhance security for high-risk installations and issued a policy that all hospitals replace gamma-based blood irradiators with x-ray technologies (Saxebøl and Øvergaard, 2013). In 2015, Norway completed its phase-out by replacing all of its 13 gamma-based irradiators and by returning the cesium-137 sources to the manufacturer.
___________________
5 Michael Itamura and Jodi Lieberman, Sandia, presentation to the committee on April 29, 2020.
In 2013, the European Radiation Protection Authorities Network surveyed European countries on their use of cesium blood irradiators and experiences with x-ray technology regarding reliability, costs, maintenance, and other factors. Within the nine countries6 that responded to the survey, there were at least 100 gamma-based and about 20 x-ray-based irradiators. Respondents mentioned that, overall, the x-ray technology worked well, but disadvantages included more breakdowns when this type of irradiator was installed in a warm area; relatively expensive equipment maintenance; and need for continuous power supply to the device with steady voltage and frequency. In addition, it was noted that x-ray-based irradiators needed a relatively long time for irradiation as compared to gamma-based irradiators, but the actual amount of additional time was not mentioned (Saxebøl and Øvergaard, 2013).
Since the 2013 survey, some European countries have made notable progress in phasing out gamma-based irradiators and replacing them with x-ray technology. By the end of 2016, France had replaced all of its 30 gamma-based cesium irradiators (Bieniawski et al., 2017). In June 2017, Denmark banned the use of gamma-based irradiators, and Finland and Sweden have been encouraging the use of x-ray technology (Dalnoki-Veress and Pomper, 2017). The IAEA notes that it no longer supplies cesium sources for blood and research irradiators to developing Member States (IAEA, 2019c).
As noted in Chapter 1, India was the first country to report use of vitrified cesium in pencil-shaped form (BARC, 2017). Bhabha Atomic Research Centre (BARC) researchers developed a borosilicate glass matrix to embed the purified cesium-137 into a non-dispersible form and then poured the glass mixture into stainless steel pencils. As of spring 2021, BARC has reported that 220 cesium glass pencils have been produced for blood irradiation and grain irradiation.7 The specific activities for the vitrified cesium forms are 2.5 Ci/g (92.5 GBq/g) and 5 Ci/g (185 Gbq/g); in comparison, the specific activity of cesium-137 chloride is 87 Ci/g (3,220 GBq/g), implying that the Indian vitrified cesium pencils cannot be used in existing blood irradiators, which would have to be redesigned to accommodate the much lower specific activity material.
Use of pathogen reduction methodologies is another promising alternative to gamma irradiation for some blood components. Although blood banks could avoid the use of gamma and x-ray irradiators for prophylaxis against TA-GvHD by employing pathogen reduction methods for platelet units, no red cell pathogen reduction method has yet been approved by regulatory bodies. Until there is a licensed method for pathogen reduction for red cells, hospitals and blood banks will still need to use gamma or x-ray irradiation. Even with regulatory approval of a red cell inactivation method, not every apheresis platelet can be pathogen reduced because units must meet volume and platelet content specifications to qualify for treatment. Therefore, reliance on gamma or x-ray devices will likely remain for the near future.
4.2 RESEARCH IRRADIATORS
Research irradiators are used in a variety of studies involving cells or small laboratory animals as well as nonbiological material. Examples include:
- Irradiation of cells cultured in vitro to provide “feeder cells” to support the growth of particular cell types;
- Irradiation of cells to provide stimulator cells for immunological reactions;
- Irradiation of cells for radiobiological investigations including cell-cycle inhibition;
- Irradiation of experimental animals to treat tumors;
- Irradiation of experimental animals to deplete or ablate the hematopoietic system and facilitate bone marrow transplantation or tumor implantation for research purposes;
- Irradiation of experimental animals to study radiation-associated disease following different doses, dose rates, and energies from the applied radiation; and
- Irradiation of materials (polymers, biologicals, food, microelectronics and other material) with different total doses, dose rates, and energies to understand the effects of radiation on these materials.
___________________
6 Belgium, Czech Republic, France, Germany (just the state of Bavaria), Luxembourg, Slovenia, Spain, Sweden, and Switzerland.
7 Kaushik, C. P. 2021. Recent Developments in Recovery of Radioisotopes by HLLW Partitioning. Indian Nuclear Society. INS Newsletter. May 2021.
Studies that use research irradiators are conducted in research institutes, universities, and government and commercial laboratories. In contrast to blood irradiators, where FDA provides the specifications for irradiated blood, the specifications in research may vary significantly depending on the experimental needs and endpoints studied.
4.2.1 Radioisotope Technologies
Research irradiators using gamma sources are similar in design to blood irradiators. The typical activity of the Category 1 and Category 2 cobalt-60 or cesium-137 radiation sources is approximately 1,200–3,000 Ci (44.4–111.0 TBq). The shorter half-life of cobalt-60 when compared to cesium-137 requires that the sources be replaced more frequently. However, the higher-energy gamma rays from cobalt-60 and the relatively high activity that can be achieved from an equivalent amount of radionuclide make it an attractive radioisotope for the delivery of higher dose rates required in some research protocols.
There are about 300 gamma research irradiators operating in the United States (CISA, 2019). Worldwide, more than 2,000 cesium research irradiators and about 500 cobalt-60 irradiators have been installed (IAEA, 2019c).
4.2.2 Alternative Technologies
The most viable alternative to cesium-137 and cobalt-60 research irradiators are x-ray irradiators. Commercially available x-ray irradiators used in research produce radiation beams with reasonable field geometry and overall dose-homogeneity (Poirier et al., 2020). These irradiators operate over a wide range of different energies, from 120 to 350 kV, to cater to the different experimental needs. In addition, x-ray irradiators produce photons on a continuous energy spectrum. Therefore, x-ray irradiation outcomes are more dependent on the peak energy, dose distribution, depth dose, filtration of beam, and other parameters of the specific x-ray equipment being utilized. The price of the irradiators increases as the voltage increases, as does the overall size of the irradiator.
Reviews have reported on the processes and results of experiments carried out to assess replacement of gamma irradiators with x-ray technologies. In general, higher-energy x-ray irradiators (220–350 kV) are more likely to make suitable replacements for carrying out animal studies, while lower-energy (160 kV) x-ray irradiators are more suitable replacements for in vitro cell studies (Murphy and Kamen, 2019). These reviews also noted that achieving a comparable relative biological effectiveness (RBE) value may be possible in some but not all cases (MacKenzie et al., 2020). Knowledge of the desired depth dose can help identify the appropriate x-ray irradiator and setup required (Murphy and Kamen, 2019). The depth dose curve for a 320-kV x-ray irradiator was nearly identical to that of a cesium-137 irradiator down to a tissue depth of 4 cm, but a 160-kV x-ray irradiator was only able to produce depth doses similar to those of cesium-137 to tissue depth less than 2 cm (MacKenzie et al., 2020).
Low-energy x-ray irradiators are disadvantageous for animal studies because of the shallow depth-dose curve and risk of skin burns. Because the use of higher-energy x-ray irradiators is necessary to attain adequate tissue penetration, appropriate energy filtration must be used to block the lower-energy photons, thereby allowing for good tissue penetration while sparing surface tissue. As mentioned in Section 4.1, Stellarray is developing a range of irradiators for isotope replacement based on a new x-ray source architecture, FPXS. This new system could offer the advantage of improved dose uniformity in research but that would need to be demonstrated in equivalency experiments.
It is generally agreed that x-ray technologies can replace cesium irradiators with some exceptions, for example, when very high radiation doses are required. Some uses of research irradiators require higher x-ray penetration with good dose uniformity and therefore higher energy. The shorter depth penetration of x-rays compared to cesium-137 will have an impact on some research studies, particularly those using experimental animals larger than mice. This issue may be practically addressed by efforts to implement x-rays of the highest possible beam energy and penetration (Poirier et al., 2020). Although linacs are an attractive alternative to gamma irradiation because they can be used to generate a field with the same mean energy, the added cost of building and operating the facility make them an unlikely alternative, leaving x-ray irradiators as the more practical option (IAEA, 2019c).
4.2.3 Alternative Technology Adoption Considerations
Many research institutes, universities, and government laboratories have begun to transition from utilizing cesium-137 and cobalt-60 research irradiators to using x-ray irradiators. As with replacement for blood irradiators, CIRP has facilitated the adoption of x-ray technology for research purposes in the United States. Since inception of CIRP, about 25 percent of research irradiators in the United States have been replaced (CISA, 2019). However, this process has faced a number of challenges, primarily because researchers must individually conduct comparison studies to demonstrate equivalency between a gamma and an x-ray irradiator or calibrate experimental parameters. They must also establish new protocols and procedures so that they can, with reasonable certainty, decide whether a particular type of x-ray irradiator is sufficiently equivalent to the irradiator they had previously been using or determine a conversion factor such as an RBE to account for variances in outcome and ensure continuity between legacy experiments and future results.
The need to achieve the same biological effect from x-ray and cesium-137 depends on the experimental question. Although in some cases it is necessary to provide for a one-to-one dose substitution, in some other cases a simple conversion factor may be sufficient (Andersen et al., 2020). Public Health England recently published a comprehensive report that includes a decision tree to help guide selection of a replacement technology for cesium irradiators for research (and blood) applications (Barnard et al., 2020; see Figure 4.4).
Along the way, researchers had to address issues relative to the biological model used and energy spectrum. In addition to the above-mentioned technical challenges, universities and research centers that consider replacing
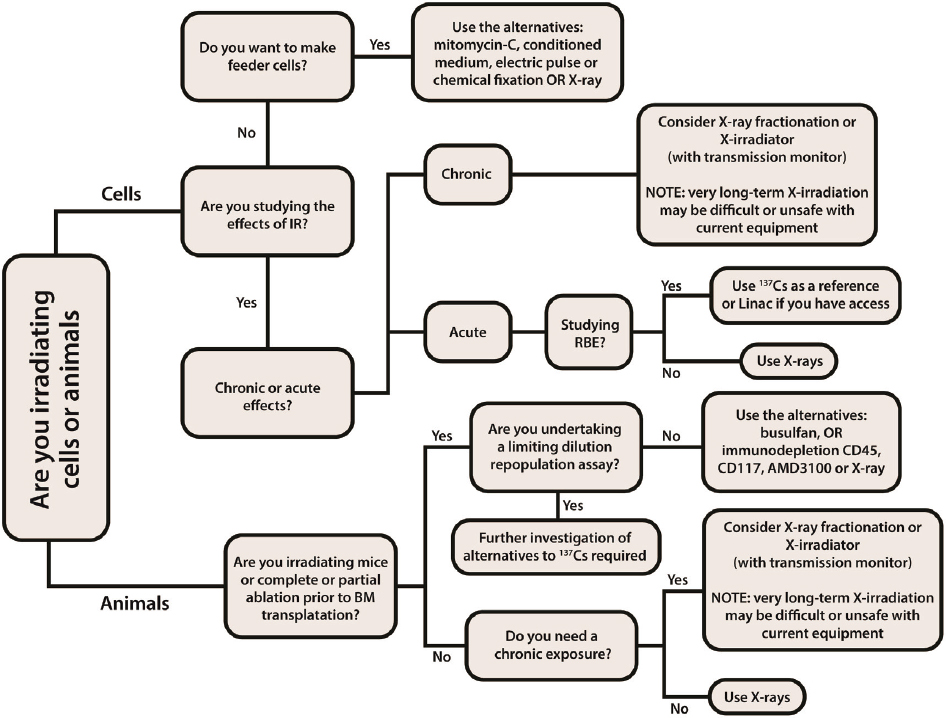
SOURCE: Adopted and revised from Barnard et al., 2020. Reproduced with permission from Public Health England and the UK Home Office Joint Security and Resilience Centre.
their cesium-137 and cobalt-60 research irradiators are often facing logistical and financial challenges, despite the incentives provided by CIRP (see Sidebar 1.2) These comparison experiments consume resources that are typically scarce in research institutions.
In assessing alternative technologies, researchers rely to some degree on relevant published comparison studies from other investigators and information from the manufacturers. However, most of these comparison studies are specific to a cell type or animal model (Afrough et al., 2020; Andersen et al., 2020; Belley et al., 2015; Gibson et al., 2015; Gott et al., 2020), and therefore extrapolating knowledge from one to a different cell type or animal model or endpoint introduces uncertainties. It can also be difficult to reproduce studies of others if experimental details are not disclosed in the description of the irradiation conditions. For example, work by Gibson and colleagues (2015) showed increased morbidity in mice exposed to low-energy x-rays (130 kVp) when compared to mice exposed to an equivalent dose of cesium-137 gamma rays. However, the researchers did not specify a number of experimental parameters including the filtration used.
Representatives from the University of California system who briefed the committee noted that a lesson from the adoption of x-ray irradiators for research was the importance of involving senior management and the researchers in the decision-making process and allowing for exceptions when equivalency of cesium-137 to x-ray cannot be established. They also pointed to the need to identify additional funding resources through the university or the hospital to support equivalency studies.8
As noted in Section 4.1, several government initiatives aimed to eliminate use of cesium-137 in commercial applications. In the 2019 Nuclear Defense Authorization Act Section 3141, Congress set an ambitious goal of phasing out all cesium blood and research irradiators in the United States by 2027 (U.S. Congress, House, 2018). An obstacle to achieving this goal is replacement of gamma irradiators used in research. However, the goal could be reached by coordination within federal agencies that typically fund research to help facilitate equivalency studies.
4.3 EXTERNAL BEAM RADIOTHERAPY
External beam radiotherapy is the most common form of radiotherapy for management of solid and hematological malignancies. It is fractionated, that is, typically delivered as a course of once-daily treatments, 5 days per week, over a period of weeks. Treatment devices that incorporate gamma-emitting sources (cobalt-60) for use in external beam radiotherapy are called teletherapy machines.
In high-income countries, there generally is sufficient access to radiotherapy, although regional and population disparities in access and outcomes do exist. There is also continuous innovation and development of new treatment technologies and shifts in practices in radiotherapy, with the goal to provide more targeted treatments that increase tumor control and spare normal tissue. Some of this technology innovation has demonstrated improved patient health outcomes, but in some cases it offers only dosimetric advantages that may not improve long-term patient health outcomes. In the United States, a high-income country with a mix of public and private, for-profit and nonprofit insurers and health care providers, decisions about delivery of radiotherapy are complex and involve financial considerations such as insurance reimbursements and return on investment. Decisions about delivery of radiotherapy also reflect patient preferences regarding the trade-offs in cancer control versus side effects and costs.
Low- and middle-income countries (LMICs) are generally inadequately equipped to address the growing burden of cancer. Greater than 50 percent of patients requiring radiotherapy do not have access to treatment, and the statistics are worse in the lowest-income countries, where the unserved population is greater than 90 percent (Zubizarreta et al., 2015). Some LMICs lack radiotherapy equipment and expertise altogether.
4.3.1 Radioisotope Technologies
Cobalt-60 is currently the only commercially available radionuclide for clinical use in teletherapy. Cobalt-60 teletherapy was invented in the early 1950s, placing the cobalt unit at the forefront of radiotherapy for many
___________________
8 Kei Iwamoto, University of California, Los Angeles, and Byron Hann, University of California, San Francisco, presentations to the committee on September 9, 2020.
years. A cobalt-60 teletherapy source is typically a welded, sealed cylindrical stainless-steel capsule containing hundreds of tiny, high-activity cobalt metal pellets. A double-welded seal is used to prevent any leakage of the radioactive material from the source container (NRC, 2008). The typical diameter of the cylindrical teletherapy source is between 1 and 2 cm, and the height of the cylinder is about 2.5 cm. These cylinders typically contain between 5,000 and 15,000 Ci (185–555 TBq) of cobalt-60 at the time of purchase, typically above the Category 1 threshold. A cobalt-60 teletherapy source provides a typical dose rate on the order of 1 to 2 Gy/min at a distance of 80 cm from the source. Often the output of a teletherapy machine is stated in RMM (roentgens per minute at 1 m) as a rough guide to the source strength (NRC, 2008). For a given activity level, a smaller source diameter yields a smaller physical penumbra, making for a sharper beam edge. The source is most often mounted isocentrically, allowing the beam to rotate around the patient at a fixed source-axis distance (SAD). Modern teletherapy machines have a SAD of 80 cm or 100 cm (see Figure 4.5).
For safety, a teletherapy unit uses one of two methods to move the device’s source from the beam “off” into the beam “on” position and back: a source in a sliding drawer and a source in a rotating cylinder. Both methods incorporate a safety feature that terminates the beam (i.e., moves the source into the “off” position) automatically in case of power failure or emergency. Some radiation will escape the unit even when the source is in the beam “off” position. This head leakage typically amounts to less than 0.01 millisievert per hour (mSv/hr) (1 millirem per hour [mR/hr]) at a distance of 1 m from the source. International regulations require that the average leakage of a teletherapy machine head be less than 0.02 mSv/hr (2 mR/hr) at 1 m from the source (NRC, 2008).
Cobalt-60 teletherapy devices are relatively inexpensive (on the order of $300,000) and simple to use. Teletherapy sources are usually replaced within one cobalt-60 half-life (5.3 years) of installation. Replacement of the sources is rather simple and is done by trained technicians, eliminating the need to transfer the machines to the manufacturer for replenishment.
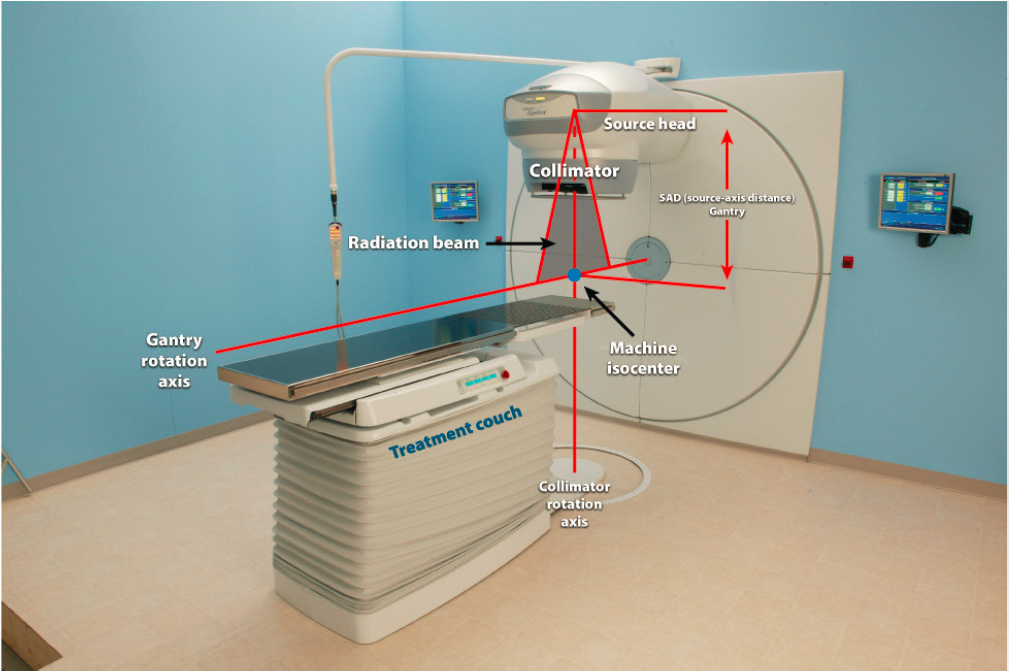
SOURCE: Best Theratronics Ltd. A TeamBest Global Company.
Use of cobalt-60 teletherapy in most high-income countries is being phased out, and there has been an almost complete transition to external beam radiotherapy using medical linacs. In the United States, there are approximately 3,000 radiotherapy centers serving a population of about 325 million. The number of teletherapy machines is small (approximately 140). In comparison, there are more than 3,400 linacs installed in these centers. In Africa, there are about 230 radiotherapy centers serving a population of more than 1.2 billion.9 There are approximately 410 teletherapy machines and 350 linacs in these centers,10 indicating that cobalt-60 teletherapy continues to be essential for radiotherapy delivery.
4.3.2 Alternative Technologies
Medical linacs are a widely used alternative to cobalt-60 teletherapy. The potential for the use of linacs in radiotherapy became apparent in the 1950s, the same time as cobalt-60 teletherapy machines were invented. Over time, the linac became the most widely used form of radiotherapy. As noted earlier in this chapter, use of linacs is standard practice in the United States and other high-income and many middle-income countries. There are approximately 3,400 linacs in the United States and around 12,300 globally, according to the IAEA’s Directory of Radiotherapy Centres. Fewer than 750 are available in LMICs according to the same directory. The International Cancer Expert Corps (ICEC) predicts that there will be a need for 5,000 to 10,000 linacs in LMICs in the next two to three decades to address the existing and expected cancer therapy needs.11
In contrast to a cobalt-60 unit that provides only two gamma rays at energies of 1.173 MeV and 1.333 MeV, a medical linac accelerates electrons to megavoltage kinetic energies ranging from 4 MeV to 25 MeV and can produce two types of radiation beams useful in external beam therapy: electron beams (e-beams) and x-ray beams. X-ray beam radiotherapy directs an accelerated electron beam toward a metal target, whereupon some of the electron’s kinetic energy is transformed into photons. These photons, referred to as bremsstrahlung x-rays, are subsequently formed into a clinical x-ray beam with the help of a flattening filter and special collimators (NRC, 2008). Linacs that produce megavoltage x-ray beams are typically used for the treatment of deep-seated lesions and can reach complicated areas such as the brain stem. Megavoltage e-beams are commonly used for the treatment of superficial (skin) lesions. A linac provides a typical dose rate on the order of 3 to 6 Gy/min.
Compared to cobalt-60 teletherapy machines, linacs are complex because of multimode capabilities that have evolved and are available on most modern machines. Complex software and hardware allow for high-dose-rate modes, multileaf collimation, electron arc therapy, dynamic collimator motion, and stereotactic radiosurgery (NRC, 2008) (see Section 4.4).
Linacs are usually mounted isocentrically, and the operational systems of the machine are distributed over five sections: gantry, gantry stand or support, modulator cabinet, patient support assembly (treatment couch), and control console (see Figure 4.6). The cost of a linac is in the range of $2 million to $4 million, significantly greater compared to cobalt-60 teletherapy machines. Infrastructure and maintenance requirements are also more demanding for linacs due to complex electric components and higher staffing and training requirements (Healy et al., 2017).
Incremental advancements in the application of linac x-ray and e-beams are being continually developed. Those that demonstrate positive clinical outcomes quickly transition to becoming “state of the art.” Some examples are intensity-modulated radiotherapy (IMRT), volumetric-modulated arc therapy (VMAT), and image-guided radiotherapy (IGRT). Each technological advancement appears to improve the precision of treatment planning and dose delivery, but the impact on patient health outcomes has been small and slow while treatment costs have escalated significantly. In some cases, there has been a marked difference between the theoretical cost-benefit improvements promoted by the manufacturers and those realized by practitioners (Bruner et al., 2015; Kale et al., 2016).
___________________
9 At least 10 African countries have no radiotherapy center.
10 Information is stored at the IAEA’s Directory of Radiotherapy Centres (DIRAC). See dirac.iaea.org.
11 C. Norman Coleman, International Cancer Expert Corps, presentation to the committee on August 18, 2020.
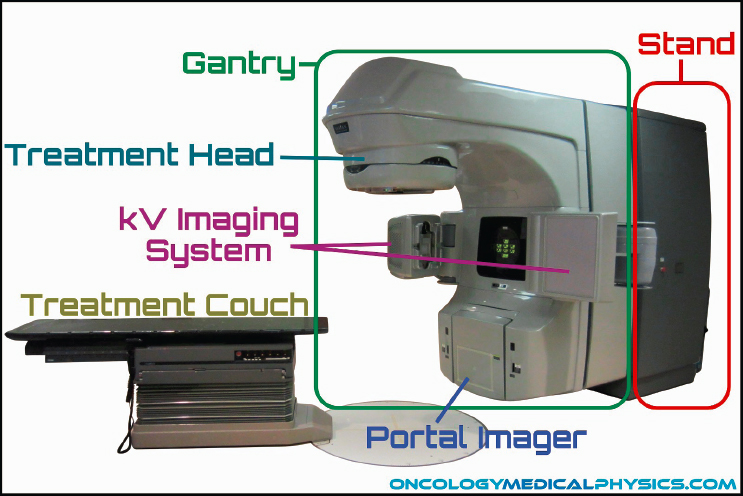
SOURCE: Image courtesy of oncologymedicalphysics.com.
Further Advancements in Alternative Technologies
Advancements in medical technologies for external beam radiotherapy continue to evolve and include new techniques and even new particles. A detailed description of the developing technologies is beyond the scope of this committee’s work because these technologies do not directly bridge the transition from cobalt-60 teletherapy utilization to nonradioisotope technologies. These developing technologies are therefore only briefly described in the following paragraphs.
Proton and ion beam therapies use high-energy positive ions (either protons or carbon ions) to treat cancer. They can more precisely deliver radiation dose to a tumor with a steep decline in energy at the tumor margins, meaning less radiation exposure to normal tissues. The RBE of particle beams is different from photon beams and requires adaptations in treatment planning (Baumann et al., 2020). Proton and ion beam therapies have advantages over photon-based therapy, especially related to the risk of secondary malignancies, which has important implications for the treatment of several cancers including pediatric cancers. However, in adults, tumor control is similar to standard radiotherapy for the same given total dose, and the treatment costs can be up to 10 times greater (Peeters et al., 2010) due to the higher cost of the equipment.
Carbon ion therapy uses high-energy heavy ions to kill cancer cells. The high linear energy transfer (LET) and other characteristics of this radiotherapy modality may have indications for tumors that have been traditionally refractory to standard photon radiotherapy. Carbon ion therapy has, in principle, improved RBE and a lower oxygen enhancement ratio over proton beam therapy. This may mean it could enhance outcomes of radioresistant tumors such as sarcoma and malignant melanoma and those with high lethality such as liver and pancreatic cancers. The downside is that the costs of treatment with carbon ion therapy are much greater than standard radiotherapy and approximately twice those of proton therapy (Pompos et al., 2016). The proportion of patients that could potentially benefit from the incremental improvements in tumor control offered by carbon ion therapy is estimated at approximately 2 percent of patients eligible for radiotherapy (Blakely et al., 2019). There are currently 13 carbon ion facilities operating around the world (Malouff et al., 2020).
A radiotherapy technique currently at early stages of development is ultra-high dose rate (FLASH) radiotherapy. First described in 2014, FLASH radiotherapy delivers radiation treatment at ultra-high dose rates (> 40 Gy/s)
compared to conventional radiotherapy. Early laboratory studies indicate that FLASH radiotherapy may further reduce normal tissue adverse effects beyond what is presently seen with current photon treatments (Favaudon et al., 2014). Clinical trials on FLASH radiotherapy were initiated in October 2020 and are still at the patient recruiting stage (NLM, 2020). The goal of the trials is to assess the workflow feasibility of FLASH radiotherapy treatment in a clinical setting, as well as the toxicities and pain relief when used to treat bone metastasis. If proven feasible, implementation of this technology will require advanced ancillary equipment such as ionization chambers, radiochromic films, and semiconductor detectors (Esplen et al., 2020).
Technology Development to Address Costs and Complexities of Linacs
At least two large companies, manufacturers of medical devices for radiotherapy, have invested in research and development of devices that could address the infrastructure challenges in LMICs that often prevent normal operation of a linac. For example, Varian recently launched Halcyon, a linac that the company says will increase global access to radiotherapy while reducing the shielding, staffing, and reliable electricity requirements, as well as cost. Experiences of users of this machine in Nigeria and Guatemala are described in Section 4.3.3.
Best Automation & Robotics, a member of the TeamBest group of companies, is developing a system to address the challenges of operating a linac in LMICs. This system’s power requirements are said to be more flexible and resilient and therefore tolerant of a fluctuating electrical supply. The committee does not have knowledge as to when this technology might become available.
Smaller companies also invest in research and development of machines that could address the infrastructure challenges in LMICs. Euclid Techlabs LLC, a small business recipient of NNSA’s SBIR award, is developing a process to improve components for the linac that would maintain quality and decrease costs. The company’s goal is to reduce costs of the linac by eliminating the brazing step (a complex melding of metals) and using stainless steel components as opposed to copper. In addition, the company aims to reduce the weight and footprint of the linac machine (see Figure 4.7). The company’s estimate for a 1-MeV turnkey system is approximately $100,000. Also at early stages of development is a second project by the same company aiming to demonstrate a 1-MeV dielectric accelerator-based suitcase x-ray source and also produce a cost-effective and compact medium energy (~4 MeV) design. An x-ray beam between 4 and 6 MeV has a penetration profile that is comparable to a cobalt-60 source. If such a system were available at a cost similar to a cobalt-60 teletherapy machine and had a flexible, resilient
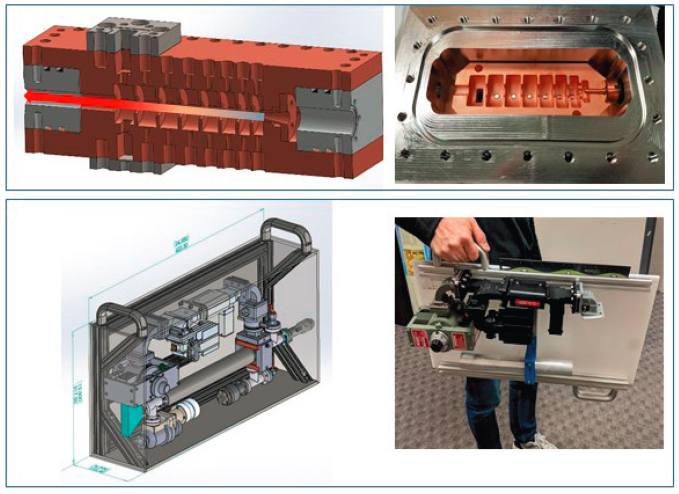
SOURCE: Euclid Techlabs LLC.
power supply, it could be a feasible replacement for teletherapy in LMICs. Both projects by Euclid Techlabs LLC are at very early stages of development and are subject to the standard challenges and uncertainties of bringing new ideas to market.
Another small business recipient of a SBIR award (TibaRay) also aims to design an affordable multi-MeV linac. The designs developed by this company include several novel elements in the cavity design, power sources, and power supplies and look to reduce the cost to manufacture and also improve the resiliency of the system in challenging operating environments. TibaRay aims to make these linacs economical for widespread adoption and use. TibaRay’s primary goal is to produce a 16-accelerator system arrayed around the patient (see Figure 4.8) to deliver treatment to the patient at a very high dose rate, equivalent to FLASH radiotherapy described earlier in the chapter and do this at a price equivalent to existing single-accelerator systems. Successful development and deployment of this device rely on significant reduction in costs for the linac components.
In addition to research and development, the U.S. government has also supported basic science research. In 2019, DOE’s Office of Science, with support from NNSA, the Department of Defense, the Department of Homeland Security, and the National Institutes of Health hosted a workshop with the overall goal to advance and deploy state-of-the-art compact accelerator technologies in the near future. Focus was given to promising accelerator technologies that can achieve a Technology Readiness Level (TRL) of 5, meaning validation in relevant environments, within the next 5 years (DOE, 2019). Workshop participants identified five key research priorities:
- Revolutionize accelerator design to produce modular, interoperable, robust systems;
- Develop “smart accelerators” that produce expected results in difficult environments;
- See beyond present technological limits;
- Control effects and outcomes beyond present technological limits; and
- Revolutionize the size to enable new and emerging applications.
These efforts by DOE’s Office of Science could lead to improved designs for linacs and further support their adoption in LMICs.
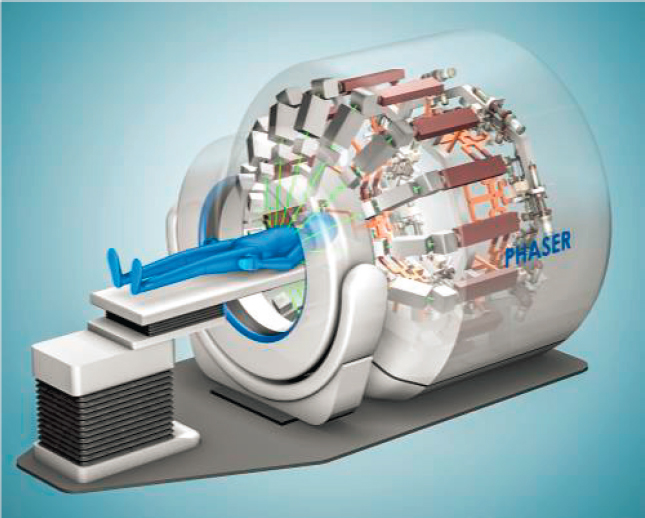
SOURCE: TibaRay.
4.3.3 Alternative Technology Adoption Considerations
As noted at the beginning of this section, the discussion of adopting alternative technologies to cobalt-60 radiotherapy is relevant to LMICs because cobalt-60 teletherapy has almost entirely been phased out in the United States and other high-income and many middle-income countries and has been replaced by external beam therapy using linacs.
Both cobalt-60 sources and linacs are clinically acceptable and have a long history of successfully treating cancer patients; however, external beam therapy using linac is considered superior to cobalt-60 teletherapy. Cobalt-60 units are simpler to use, less dependent on infrastructure resource requirements, and reportedly have less machine downtime. However, treatments using cobalt-60 are typically longer due to lower dose rates compared to linacs. Linacs offer better localization of the radiation dose to the tumor and limit damage to adjacent tissues. They are generally more expensive and more complex machines, have higher requirements for skilled workforce, and higher demand for maintenance, which can result in prolonged downtimes and high service costs. They also require a reliable supply of electricity, which is an issue in many LMICs. A number of African countries have reported several hours of electric outages in a typical month. Nigeria reported the highest number (about 33 hours in 2015). Countries in Asia also reported a high number of hours of electric outages. For example, Pakistan reported 72 hours in 2013, and India reported 14 hours in 2014. Except for one country (Guyana, 8.5 hours in 2010), countries in Central and South America reported less than 3 hours of electric outages (see Figure 4.9).
Despite the recognized sophistication of linac systems, it has been argued that cobalt-60 teletherapy may be an appropriate solution for radiotherapy in LMICs (Healy et al., 2017). Others have argued that for radiological safety and security reasons, linacs are the most effective solution to the radiotherapy needs in LMICs (Coleman et al., 2017).
The almost complete adoption of linacs in high- and many middle-income countries has created gaps in cobalt-60 teletherapy technology advances. Some features of linacs, such as multileaf collimators, dynamic wedges, and dynamic operation, can also be installed on cobalt-60 teletherapy machines to improve treatment. However, in the absence of a market in high- and many middle-income countries, manufacturers of cobalt-60 machines have
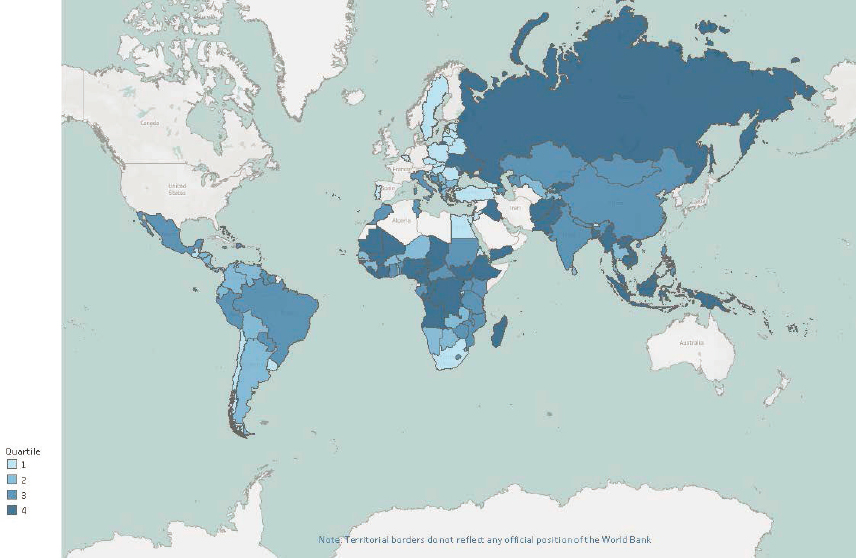
SOURCE: World Bank Enterprise Surveys, 2021. See https://www.enterprisesurveys.org/en/graphing-tool.
been slow in adopting these modern features, conceding preeminence to linac manufacturers even in parts of the world that would find it much easier and more practical to operate cobalt-60 teletherapy machines (NRC, 2008).
In addition to technological delays, the almost complete adoption of linacs in high- as well as many middle-income countries has resulted in a disregard of cobalt-60 teletherapy as a treatment option by professional radiation oncology organizations that develop and update guidelines for use and safety of radiotherapy units. This focus on more advanced but also significantly more resource-intensive treatment modalities has disadvantaged how cobalt-60 teletherapy is viewed, even in low-income countries where teletherapy may be the only viable radiotherapy modality to treat people with cancer. In addition, today, only a small number of medical professionals receives training on operating teletherapy machines, which could result in a skill set gap in the near future.
The IAEA has several programs that aim to assist LMIC Member States with access to radiotherapy by supporting decisions in selecting and purchasing radiotherapy machines, machine setup, and training. For example, in 1990, the IAEA established the African Regional Cooperative Agreement for Research, Development and Training related to Nuclear Science and Technology (AFRA). AFRA is an intergovernmental agreement established by African Member States to strengthen and enlarge the contribution of nuclear science and technology to socioeconomic development on the African continent. The IAEA also established the Programme of Action for Cancer Therapy in 2004 to work toward the integration of radiotherapy into comprehensive cancer control plans and to engage with other international organizations such as the World Health Organization (WHO) to address cancer control in a comprehensive way. The IAEA works closely with WHO, the International Agency for Research on Cancer (IARC), the Union for International Cancer Control (UICC), and many other organizations to address challenges of cancer care in low- and middle-income Member States. The IAEA also consults on radiation safety and security with LMICs, although funding for these activities is a challenge.
Case Studies: Transition of Cobalt-60 Teletherapy to Linacs in LMICs
The committee received information from representatives of African and Latin American countries to better understand the current status of adopting linacs for radiotherapy in Ethiopia, Ghana, Guatemala, Nigeria, Senegal, and Zambia. Summaries of the information received are presented in the following sections. These case studies provide insights into the difficulties in balancing a desire to adopt more sophisticated cancer therapy technologies and migrate to safer technologies in terms of security risks with the need to maintain radioisotope technology to ensure adequate medical treatment for people with cancer in LMICs. Specifically, the case studies highlight the following challenges with adopting linac technologies in LMICs:
- Linacs are frequently purchased or deployed prior to completing facility modifications and personnel training and are stored unused in some cases for years;
- The most relevant stakeholders (i.e., medical professionals, including medical physicists) are not always involved in the decision-making process to ensure that machine specifications meet operational needs;
- Lack of reliable power supply can lead to long downtimes for the linacs and therefore delays in patient cancer treatments;
- Lack of a sustainable pathway for equipment repair and maintenance leads to even longer downtimes for the linacs; and
- Cobalt-60 teletherapy machines that are replaced by linacs are not always immediately disposed of.
Based on these case studies, it is likely that at least for the foreseeable future, cobalt-60 teletherapy will play an important role in cancer therapy in LMICs due to the lower cost, simplicity of design, reliability, and ease of operation.
Ethiopia
Ethiopia has one operating cobalt-60 teletherapy machine that serves a population of 105 million. In 2009, the government of Ethiopia, in collaboration with the IAEA, initiated a national project aimed at expanding radiotherapy and nuclear medicine services in the country. As part of the national project, the government purchased
six linacs for six participating medical centers. Linac selection and purchase decisions were made solely by the Ministry of Health, without consulting with oncologists and medical teams and other experts including the IAEA. Although governmental leadership may have initiated good-faith efforts to bring the most up-to-date technology to its citizens, it was done without considering the realities of the workforce and other resource infrastructure needs required to operate linacs, such as reliable electricity supply. The committee received information from one of these centers, the Black Lion Hospital in Addis Ababa, the capital and largest city of Ethiopia. Black Lion received its first linac in 2018, but attempts to commission it are still ongoing, primarily due to lack of appropriate personnel training in anticipation of its installation. Furthermore, there were difficulties with the vault construction that would securely house the machine, and as a result the machine and parts remained in shipping containers for 2 years prior to installation. In addition to these challenges, commissioning of the linac in Ethiopia is affected by frequent power outages in the country. These outages result not only in downtime for the machine but also in significant additional time required to restart it and check its output. The hospital plans to purchase a generator to assist with the power outage problem. The experts in Ethiopia who provided the information to the committee suggested that retaining the cobalt-60 teletherapy machine is important to ensure that patients can receive radiotherapy for cancer treatments.
Ghana
Ghana has five operating radiotherapy machines (three linacs and two cobalt-60 machines) located in three radiotherapy centers that serve a population of 29 million. Adoption of linacs started in 2008 when the OPEC Fund for International Development approved a $14.3 million loan to the government of Ghana to expand radiotherapy centers at the Korle Bu Teaching Hospital (KBTH) at Accra, and the Komfo Anokye Teaching Hospital (KATH) in Kumasi. The expansion involved constructing an additional building at each hospital to house a full range of modern diagnostic and cancer treatment equipment, including linacs. The linacs were purchased and delivered in 2012, but the building that would house the linacs was not completed until 2016 at KBTH and 2019 at KATH. As a result, the linac components remained in boxes in the corridors of the oncology department for years. At KBTH, power outages caused components of the linac to fail and additional delays in its commissioning. Treatments finally started at the third quarter of 2020 (KBTH, 2020).
Guatemala
Guatemala has six linacs located in four radiotherapy centers that serve a population of about 17 million. The School of Medicine at Washington University in St. Louis partnered with Liga Nacional Contra El Cancer (LIGA)/Instituto Nacional De Cancerologia (INCAN) in Guatemala to help modernize the country’s radiotherapy. The institute treats about 140 cancer patients per day, mostly with gynecological cancers. The center currently has three linacs; two were introduced in 2014 and the third in 2019. The latest linac is a Varian Halcyon, and it is currently the most used (about 80 percent of the patients seen monthly). Adoption of Halcyon has been successful due the commitment of the various entities involved (Velarde et al., 2020):
- U.S. Agency for International Development/American Schools and Hospitals Abroad (USAID/ASHA) provided the grant to purchase the Halcyon;
- Varian provided the machine at a reduced cost and supported the removal of the cobalt-60 source from the designated bunker, linac installation, commissioning, and training;
- LIGA/INCAN supported the conversion of an existing cobalt-60 treatment room to house the linac system and obtained regulatory approvals; and
- Washington University in St. Louis supported the removal of the cobalt-60 teletherapy machine and provided technical support and training.
The grant application to USAID/ASHA was submitted in May 2017, ahead of the Halcyon’s market release. The grant was awarded in summer 2018, and the removal of the cobalt-60 machine from the bunker began in
April 2019. Halcyon treatments began in November 2019 and resulted in increased treatment capacity and reduced waiting times from 3–9 months to 2 weeks. Even after the conclusion of the USAID grant (in October 2020) that officially supported the collaboration between Washington University in St. Louis and LIGA/INCAN, experts from the two organizations continue to engage in weekly calls to discuss remaining challenges and new initiatives.
In contrast to many African countries, Guatemala has a reliable power supply. The anticipated challenges with Halcyon and perhaps linac in general are maintenance costs due to high-cost hardware and software. Participants noted that maintenance of linac in low-resource countries needs to be affordable to achieve sustainability of high-quality radiotherapy. An additional issue is disposal of disused cobalt-60 teletherapy sources. The institute has been working with NNSA for about 18 months on the paperwork needed to repatriate two of the three sources and dispose of them via the Off-Site Source Recovery Program. It is expected that the two sources will be removed in 2021. The third cobalt source will eventually be disposed of in Guatemala. NNSA will assist with the decommissioning and removal of that cobalt-60 source as well.
Nigeria
Nigeria currently has nine radiotherapy machines (six linacs and three cobalt-60 machines) located in six radiotherapy centers that serve a population of 190 million. The committee requested information from a contact at the National Hospital in Abuja on the experience with adopting Halcyon, a linac that (according to its manufacturer, Varian) has the potential to increase global access to radiotherapy while reducing the shielding, staffing, electricity requirements, and cost. The Halcyon machine is at the NSIA-LUTH Cancer Centre (NLCC) in Lagos and was purchased with assistance from the Intervention Fund of the Federal Government of Nigeria in 2018. Installation was completed about 18 months later. The main drivers for purchasing this specific machine were that it could be fitted in the existing bunker for the hospital’s cobalt-60 teletherapy machine and would offer high-throughput, image-guided radiotherapy. The hospital has not experienced any major issues with the machine and no extensive downtime was reported.
Senegal
Senegal has four radiotherapy machines (four linacs) located in three radiotherapy centers that serve a population of 16 million. Senegal had relied on a single cobalt-60 teletherapy unit until 2016, when it broke down, leaving an entire population with no treatment options. Following this breakdown, public debate on the lack of access to radiotherapy services and expensive treatment abroad became a national issue. This eventually triggered government action and the decision to purchase three linacs. End users were involved and consulted to ensure the machine specifications met operational needs. Others involved in the transition process were the Ministry of Health, the regulatory authority, the finance ministry, the hospital, and international organizations. Patient treatment with the linacs commenced in March 2018.
Despite the smooth transition to linacs in Senegal, the country faces challenges that prevent its similarly smooth operation. These include the prohibitive cost of the linac equipment and the high-cost maintenance contracts needed to keep the linacs operational.
Zambia
Zambia has three radiotherapy machines (one linac and two cobalt-60 teletherapy machines) located in a radiotherapy center that serves a population of 17 million. The government of Zambia commissioned the Cancer Diseases Hospital in Lusaka, Zambia, in 2007 and purchased one cobalt-60 teletherapy machine and one linac. The second cobalt-60 teletherapy machine was installed in 2015. Neither the IAEA nor other agencies with experience with adopting linac technology and knowledge of the linac market were involved in the purchase. As a result, according to one expert who provided information to the committee, Zambia may not have negotiated the best possible service contract for the linac. Depending on the severity of the problem, the linac operation could be interrupted from 2 weeks (minor issues) to 6 to 8 weeks (more severe issues). In 2019, the linac’s uptime was
80 percent. The hospital plans to phase out cobalt-60 teletherapy in about 5 years. Zambia plans to expand radiotherapy services to other regions of the country in the near future.
4.4 STEREOTACTIC RADIOSURGERY
Despite the transition from cobalt-60 teletherapy to linacs for external beam therapy, many radioisotope-based devices are still in use for a subset of radiotherapy known as stereotactic radiosurgery (SRS). SRS is a form of radiotherapy that focuses high-power energy to treat small tumors of the brain and other brain abnormalities, such as blood vessel formations and a variety of neurosurgical conditions including epilepsy. When tumors lower down in the spine or other parts of the body are treated, the technique is often referred to as stereotactic ablative radiotherapy/stereotactic body radiotherapy (SBRT). SRS is not surgical in the traditional sense because there is no incision. Instead, it uses 3D imaging to target high doses of radiation to the affected area.
SRS for the treatment of brain tumors and metastases has survival outcomes similar to those of conventional radiotherapy, but some evidence exists of incremental improvements in post-treatment neurocognitive function. However, treatment-related complications such as symptomatic radiation necrosis is thought to be higher than for conventional radiotherapy (Lawrence et al., 2010; Milano et al., 2020; Remic et al., 2020).
4.4.1 Radioisotope Technologies
Gamma Knife®, manufactured by Elekta, is a gamma-based technology that delivers radiation during SRS for treating brain tumors and other functional abnormalities. Gamma Knife® contains about 200 cobalt-60 sources. A newly loaded Gamma Knife® has a total activity on the order of 6,000 Ci (222 TBq) and delivers treatment with a dose rate of about 3 Gy/min at a 0.3-mm accuracy. This dose rate will decrease as the cobalt-60 sources decay over the half-life of approximately 5.26 years.
Gamma Knife® is a gantry-designed system wherein patients lie on a bed that slides into the machine. Once the patient is aligned, the cobalt-60 sources that are arranged around a single isocenter, treat the target area from multiple angles simultaneously and deliver highly conformal dose distributions. Patients are traditionally fitted with a mounted head frame for the treatment procedure to help direct the beams at the exact treatment location and spare healthy surrounding tissue (see Figure 4.10a). One of the newest systems, Leksell Gamma Knife®, offers both frame-based and frameless setup options (see Figure 4.10b and c) allowing for greater treatment flexibility. Varian Medical Systems and Huiheng Medical, Inc. also manufacture devices that deliver gamma-based SRS. The cost of a gamma-based SRS device is about $3 million to $4 million (Capala et al., 2015) with additional setup costs of $1 million to $2 million related to the shielding and site preparation. Maintenance costs are also high, about $10,000 for each unit annually. The expected useful life for a gamma-based SRS device such as the Gamma Knife® is about 10 years.
A recent review estimates that there are 113 gamma-based SRS devices in the United States (Dean et al., 2019), a small increase from about 104 approximately 10–15 years ago (NRC, 2008). As with the other radiotherapy devices discussed, there are disparities in access. There is one gamma-based SRS device available for 3 million to 15 million people in high-income countries but only 1 in 132 million to 370 million people in LMICs (Fezeu et al., 2014).
A Chinese company, GammaStar® Medical Group, Ltd., sells a cobalt-60 SRS device called Gyro Knife, which contains 190 sources with a total activity of 6,000–7000 Ci (260 TBq). This device is the functional equivalent of the isocentric linac dedicated to SRS, described in Section 4.4.2. The Gyro Knife therapy head pivots the source on an axis, using a multileaf collimator to direct the radiation beam at the tumor, while the gantry rotates the therapy head around the patient’s body (NRC, 2008). Unlike Gamma Knife®, which is used for treatment of brain tumors and other brain functional abnormalities, this device can be used to treat tumors at any part of the body. Another device, GammaPod, manufactured by Xcision Medical Systems, aims to deliver SBRT to treat breast cancer. GammaPod, which received FDA clearance in 2017, uses thousands of focused beams of radiation from 36 rotating radioactive cobalt-60 sources.12
___________________
12 See https://www.fda.gov/news-events/press-announcements/fda-clears-stereotactic-radiotherapy-system-use-treating-breast-cancer.
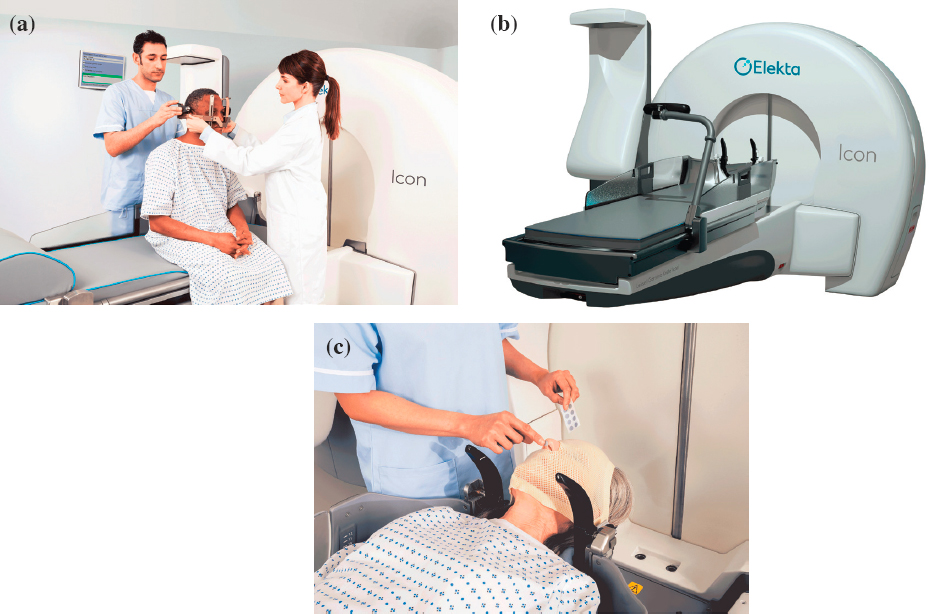
SOURCE: Elekta.
4.4.2 Alternative Technologies
There are currently three options for nonradioisotope alternatives to gamma-based SRS: SRS with standard isocentric linac; SRS with dedicated isocentric linac; and miniature linac on a robotic arm (CyberKnife®).
SRS with a standard isocentric linac can be used for the treatment of tumors anywhere in the body. The required modifications to a standard linac for use in SRS have been described in detail elsewhere (NRC, 2008).
Linacs dedicated to SRS provide clinical outcomes similar to those of Gamma Knife®. Some linacs use stereotactic frames whereas others (e.g., Novalis®, BrainLab) use a mask for immobilization and online x-ray imaging of internal structures (NRC, 2008). These sophisticated frameless techniques use a micromultileaf collimator for shaping of irregular fields and allow intracranial as well as extracranial stereotactic irradiation, with equipment and operating costs similar to those of Gamma Knife®. The price for a 15-MeV linac dedicated to deliver SRS is estimated at $2 million (Pannullo et al., 2019).
The CyberKnife® SRS system provides a different approach to image-guided dose delivery. It utilizes 6-MeV photon beams produced by a compact linear accelerator mounted on a robotic arm. The robotic arm has six degrees of translational and rotational freedom for spatial beam introduction (NRC, 2008). Stereotactically, CyberKnife® relies on IGRT for tracking its target(s) both in space and time. Therefore, the CyberKnife® allows frameless SRS, that is, it can operate without a rigid and invasive stereotactic frame improving patient comfort (see Figure 4.11). The device directs the radiation beam into the target from 1,300 positions with a reported dose-delivery accuracy on the order of 1 mm. The CyberKnife® can deliver radiosurgical dose to extracranial targets, such as the spine, lung, and prostate, by using the body skeleton or surgically implanted fiducial markers as a frame of reference for targeting purpose (NRC, 2008). The cost for a CyberKnife® is around $3–$4 million and the setup costs are around $0.5–$0.75 million (Pannullo et al., 2019). The estimated useful life of a CyberKnife® is about 10 years.
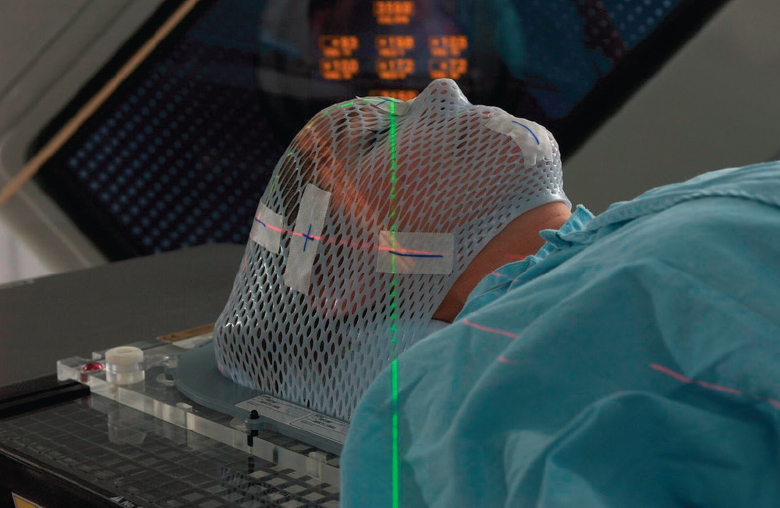
SOURCE: With permission from Mayo Foundation for Medical Education and Research. All rights reserved.
At least one company (ZAP Surgical Systems, Inc., San Carlos, California) is developing a lower-cost 2.7-MeV linac system dedicated to SRS with the intention to reduce setup costs and expand access in lower-resource countries (Weidlich et al., 2017). The system, labeled as Zap-X, is marketed as self-shielded and therefore does not typically require a radiation bunker. The system is limited by design to deliver SRS to the brain and head and neck tumors.
4.4.3 Alternative Technology Adoption Considerations
There is debate on the relative merits of Gamma Knife® versus linac-based SRS. Clear benefits of linac-based SRS are that it does not use radioactive material; it is more versatile and can be used for treating a number of tumor sites; and the costs for treatment are lower both when standard or dedicated linac systems are used (Griffiths et al., 2007). Continuing innovation will likely reduce the costs of purchasing, installing, and maintaining the linac-based SRS systems. Despite these benefits, the accuracy of delivering radiation for treatment of both single as well as multiple intracranial lesions using Gamma Knife® remains superior to any linac-based SRS system (Ma et al., 2014).
A recent retrospective study looking at practice patterns of gamma-based SRS versus linac-based SRS use in the United States by facility type, location, and other factors found that although gamma-based SRS is the most commonly used modality for single-fraction SRS, linac-based SRS use is on the rise. The study attributed the increase use of linac-based SRS to availability (specifically in community medical centers as opposed to academic medical centers) and location (in non-western states compared to western states). The authors also noted a lack of data to allow comparison of the two treatment modalities in terms of efficacy and toxicity. The choice of Gamma Knife® versus linac-based SRS in the United States is to some extent based on the condition being treated, but also on nonclinical factors such as user preference, availability, and cost (Park et al., 2016).
In just more than a decade (from 2007 to 2017), there was a greater than fourfold increase in SRS units in the United States. This increase is primarily due to broad adoption of linac-based SRS systems. By 2017, there were approximately 428 dedicated SRS systems with linac-based systems among the most common (39 percent), followed by CyberKnife® (35 percent) and Gamma Knife® (26 percent) (Dean et al., 2019). Similar trends in broader adoption of linac-based SRS systems compared to gamma-based SRS have been observed in Europe.
Overall, in the United States there is 1 SRS installation per 800,000 people, as compared to 1 per 4.1 million in Europe, and 1 per 35 million people in South America (Pannullo et al., 2019). According to the manufacturer, in Africa, there are five Gamma Knife® devices and no linac-based SRS installations in the entire continent for a population of 1.2 billion.
The inadequate numbers of neurosurgeons, radiation oncologists, and physicists in LMICs and the high costs of SRS installations represent significant barriers to solving the scarcity of SRS installations relative to patients. These barriers are enhanced by the costs of replenishment (for gamma-based SRS), maintenance, and repairs, which require expensive maintenance contracts with the manufacturers.
One group suggested that linac-based SRS has the potential to more easily be adopted by LMICs due to the economics and relative easiness to prepare the patient and operate the devices compared to Gamma Knife®. Although a team of highly skilled neurosurgeons, radiation oncologists, and physicists is still required, the group suggests that the experts can provide support remotely, allowing for less specialized local care providers to deliver on-site services. This arrangement is similar to other models of telemedicine already in place (Datta and Rajasekar, 2004).
4.5 HIGH-DOSE-RATE BRACHYTHERAPY
Brachytherapy is a form of radiotherapy in which radiation is delivered to tumor sites internally, via a catheter, also referred to as an applicator. Traditionally, brachytherapy has been delivered with radioactive material that can be categorized as permanent or temporary and as low dose rate (0.4 to 2.0 Gy/hr), medium dose rate (2 to 12 Gy/hr), or high dose rate (HDR, greater than 12 Gy/hr) depending on the technique and radionuclide selected. HDR is only used with remote afterloaders; that is, the catheter is placed first and the radioactive sources are inserted (loaded) later. Commercially available HDR systems typically treat at a dose rate of 100 to 300 Gy/hr and must be housed in well-shielded rooms.
Implementation of three-dimensional imaging through computed tomography (CT) or magnetic resonance imaging has improved planning for brachytherapy and has made it a highly accurate and reliable treatment option for many cancer types, especially gynecologic cancers such as cervical and vaginal cancers (Holschneider et al., 2019; WHO, 2014). In addition, HDR brachytherapy is an effective treatment for breast cancer when partial breast irradiation is indicated (Shah et al., 2018). The main advantage of HDR brachytherapy compared to conventional radiotherapy is that risk of side effects is minimized due to the targeted and precise nature of delivering the radiotherapy from inside the body.
Utilization of HDR brachytherapy has declined since the early 2000s (Gill et al., 2014; Han et al., 2013; Mahmood et al., 2014) in the United States and elsewhere where external beam technologies such as IMRT, SBRT, and proton therapy increasingly replace it. This deviation from established treatment guidelines (Chino et al., 2020) has been attributed to several possibilities including higher reimbursement rates with IMRT and SBRT; decreased access to HDR brachytherapy due to changing referral patterns; and resulting insufficient training of radiation oncology residents and maintenance of brachytherapy skill sets due to lower referrals. The decrease in utilization of HDR brachytherapy in treating cervical cancer has raised concerns because it has been linked to reduction in overall survival from this cancer (ASTRO, 2019).
4.5.1 Radioisotope Technologies
The most common radioisotope used in HDR brachytherapy is iridium-192. Depending on the type of cancer being treated and its location, one or more thin plastic catheters are placed interstitially or intracavitarily next to the tumor. Through computer control and based on a specific treatment plan, small radioactive pellets or seeds are pushed through the catheters to the tumor where they deliver the radiation for a specific period of time, typically 5 to 20 minutes. Once the timed treatment is completed, the radioactive sources and catheters are removed. Though most HDR brachytherapy requires a few sessions to deliver the entire prescribed treatment, it is typically performed in an outpatient setting and the patient leaves the facility between cycles.
Compared to cobalt-60 and cesium-137, which have been used for brachytherapy in the past, iridium-192 has a high specific activity, which allows for smaller source for the same activity and lower photon energy, which
requires less shielding. A disadvantage of iridium-192 is its shorter half-life (74 days), which necessitates source replenishment every 3 to 4 months. In contrast, a cobalt-60 source can be used for up to 5 years, which means that during the recommended useful life of a single cobalt-60 source, approximately 20 source exchanges of iridium-192 would have to be performed. HDR sources can only be replenished by specially trained personnel. In some countries, customs paperwork and source shipment clearances can cause significant source replenishment delays, leading to decay of a large proportion of the useful activity of the source (Mailhot Vega et al., 2018).
In the United States, typical remote afterloaders use an iridium-192 source of 10 Ci (370 GBq), and therefore HDR brachytherapy sources are a U.S. NRC Category 3 source. However, elsewhere, HDR brachytherapy sources are considered Category 2 sources based on the IAEA categorization system.
4.5.2 Alternative Technologies
Electronic brachytherapy offers several unique features compared to traditional brachytherapy, including lower energy (typically less than 120 kVp) photons compared to isotope-based treatment and therefore reduced shielding needs. However on the basis of available data, the American Brachytherapy Society stated that “use of electronic brachytherapy outside of prospective registry or trial is not recommended for patients with breast cancer, nonmelanomatous skin cancers, or patients with endometrial or cervical cancer requiring vaginal cuff brachytherapy” (Tom et al., 2019).
There are currently three devices capable of electronically generating intracavitary brachytherapy; the Xoft Axxent system (Xoft/iCAD Inc.), the INTRABEAM system (Carl Zeiss Meditec), and Papillon (Ariane Medical). These systems use x-rays rather than radionuclides to generate therapeutic radiation. Each system has unique characteristics: the Xoft system is used for intracavitary and surface brachytherapy; the INTRABEAM is most often used in intraoperative brachytherapy; and the Papillon can be used for rectal, intraoperative, and superficial treatments. A comprehensive review of the different systems was authored by Eaton (2015).
The Xoft Axxent system, first released in 2006, uses a miniature x-ray tube on the end of a cable, used to carry power and cooling water to the x-ray tube that is positioned in the patient (see Figure 4.12). Each x-ray tube system has a limited life, estimated to be 12 hours or 30–50 treatments, after which it must be replaced. The system comes with various applicators that are used for specific treatment areas (e.g., uterus, cervix, endometrium). It is designed to be used in an outpatient setting, much like conventional HDR brachytherapy. One small clinical trial
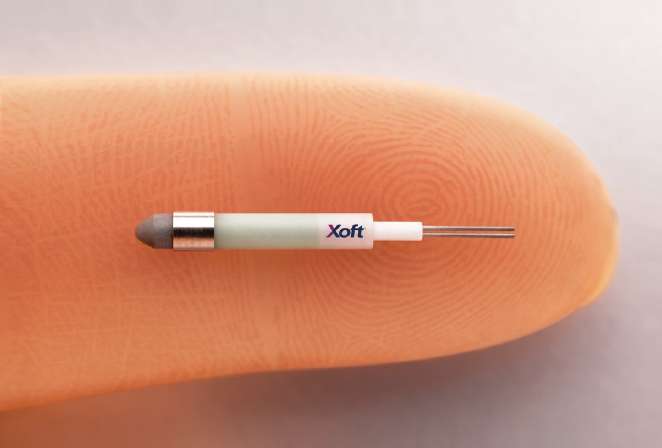
SOURCE: Courtesy of Xoft Inc., a subsidiary of iCAD, Inc.
showed promising clinical results using the Xoft system for electronic brachytherapy to treat cancers in locations where traditional HDR may not be an option (Lozares-Cordero et al., 2019).
Intraoperative radiation therapy (IORT) from INTRABEAM enables targeted treatment of certain tumors during surgery. Using low-energy x-rays, intraoperative radiation can be given with a high dose precisely into the tumor or, after tumor resection, into the remaining tumor bed. In the United States, INTRABEAM has FDA 510(k) clearance13 specifically for IORT of tumors in the brain, breast, gastrointestinal system, head and neck area, spinal metastases, and certain skin cancers.
The INTRABEAM system has a rigid channel between an electron accelerator and the target that is positioned in the patient. An electron gun emits electrons into the accelerator section. The beam (max energy 50 keV) passes through a deflector that steers it toward a gold target and creates a spherical dose distribution at the end of the line. The INTRABEAM system can be used intracranially and for head and neck lesions using a close-fitting sterile catheter and also for breast lesions using a spherical applicator. Because the INTRABEAM treatment is intraoperative, it is not a viable alternative to the most common uses of HDR brachytherapy, which are to treat gynecological cancers in an outpatient clinic setting.
The Papillon system has been used to treat rectal and superficial (skin) cancers as well as to deliver intraoperative brachytherapy. Similar to the INTRABEAM, the Papillon is not currently able to treat gynecological cancers, making it an unsuitable direct replacement for HDR brachytherapy.
4.5.3 Alternative Technology Adoption Considerations
Gynecological cancers, including cervical cancer, present a significant health burden in LMICs. The inability of patients to receive treatment is reflected in the very low 5-year survival rates for cervical cancer in Africa (15 to 30 percent) as compared to rates in higher-income countries (60 percent in North America; Denny and Anorlu, 2012). Because of the low number of HDR brachytherapy systems in LMICs and the high demand for such resources, the potential for increases in the number of HDR-brachytherapy systems in these countries is likely in the near future (Grover et al., 2015). The IAEA has also recognized the need for additional HDR brachytherapy systems worldwide (IAEA, 2015a,d).
Some African countries are implementing new brachytherapy programs, and others are expanding existing brachytherapy infrastructure. Radiating Hope, a nonprofit organization whose mission is to provide radiation therapy equipment to underresourced countries, provided an HDR brachytherapy unit in 2013 to the Institut Curie’s cancer center in Dakar, Senegal, which enabled thousands of Senegalese women with cervical cancer to receive treatment (Einck et al., 2014). Numerous challenges had to be overcome, including lack of dedicated space to install the equipment, lack of a CT simulator in close proximity, inability to perform conscious sedation in the treatment room, and inability to get the treatment planning system to work on the existing computer infrastructure. Ultimately, through concerted innovative thinking, a series of solutions was developed so that the HDR system could be used.
Manufacturers of brachytherapy systems such as Elekta and Varian recognize the need to expand brachytherapy capacity and have developed initiatives toward that goal. Elekta has developed a program called BrachyAcademy,14 which aims to advance the use of brachytherapy worldwide. Similarly, Varian is playing an active role in Cervix Cancer Research Network, a subsidiary of the Gynecologic Cancer InterGroup, which is focused on increasing access to clinical trials to improve outcomes for women with gynecological cancers in LMICs.
Despite these efforts, there are challenges associated with HDR brachytherapy. These include expense of room shielding, requirement for frequent source changes, and requirement for specific training and knowledge of the afterloader’s components. This is particularly relevant in LMICs, where technical expertise is not always available. The Xoft system has potential for becoming a viable alternative to iridium-192 HDR brachytherapy in low-resource countries, because it does not require a bunker and it is mobile. Caveats of the Xoft Axxent system relate to the ongoing cost for the replacement of the x-ray tube system on a daily basis in a busy clinic and the limitations to the types of gynecological cancers that can be treated based on the available applicators.
___________________
4.6 CHAPTER 4 FINDINGS AND RECOMMENDATIONS
Finding 11: The most notable progress with adopting alternative technologies is the worldwide adoption of x-ray technologies for blood and research irradiation. In the United States, the financial incentives provided by the government through the Cesium Irradiator Replacement Project are a major contributor to the transition from cesium irradiators to x-ray technologies and the gradual phasing out of cesium-137 in the form of cesium chloride from medical and research applications. Additional progress could be made with replacement of cesium irradiators used in research by assisting the research community with designing and funding equivalency studies.
X-ray blood irradiators are recognized as a suitable and economic alternative to cesium-137 and cobalt-60 irradiation. Broad adoption of x-ray irradiators for blood irradiation followed the implementation of CIRP. This project provides financial incentives toward the purchase of a new nonradioisotopic device as well as the removal and disposal of the cesium-137 and cobalt-60 irradiator. To date, CIRP has helped remove 165 cesium-137 irradiators, and another 150 irradiators are being scheduled for removal. Broad adoption of x-ray technology for blood irradiation has also taken place elsewhere.
Similar to replacement of blood irradiators, CIRP has facilitated the adoption of x-ray technology for research purposes in the United States. Since inception of CIRP, about 25 percent of the research irradiators in the United States have been replaced. However, this process has faced challenges, primarily because researchers must individually calibrate experimental parameters and establish new protocols for the alternative irradiation modality. This way, they can—with reasonable certainty—decide whether a particular type of x-ray irradiator is of sufficient equivalency to the irradiator they had previously been using or to determine a weighting factor to describe differences in outcome and ensure continuity between legacy experiments and future results. These comparison studies consume resources that are typically scarce in research institutions.
Recommendation F: The National Nuclear Security Administration should engage with federal partners such as the Department of Health and Human Services, the National Science Foundation, and the Food and Drug Administration to support equivalency studies for researchers who are considering replacing their cesium or cobalt research irradiators with alternative technologies. The findings of these studies should be made broadly available.
In the 2019 Nuclear Defense Authorization Act (Section 3141), Congress set an ambitious goal of phasing out all cesium blood and research irradiators in the United States by 2027 (U.S. Congress, House, 2018). An obstacle to achieving this goal is replacement of cesium-137 and cobalt-60 irradiators used in research. However, the goal could be reached by coordination within federal agencies that typically fund research to help facilitate equivalency studies. NNSA and federal partners could support researchers with adopting alternative technologies by awarding grants to support equivalency studies; funding collaborative projects between national laboratories and industry aiming to collect comparison data (see Section 5.2.3 for discussion of the Team Nablo project that could be used as a model for comparison studies); defining substantial evidence for proof of equivalency to help guide researchers; and streamlining processes for evaluation of evidence in an application that involves equivalency decision.
Finding 12: Alternative technologies do not provide a “one-size-fits-all solution,” and this is particularly evident in medical applications across high- and low- and middle-income countries because of the stark disparities in access to health care and resources. Adoption of alternative technologies for cancer therapy in some low- and middle-income countries has had unintended negative impacts on patient care because of lack of a trained workforce, required resources, and infrastructure to make these alternatives viable options.
Medical linacs are a widely used alternative to cobalt-60 teletherapy units and are the standard practice in the United States and other high-income and many middle-income countries. Compared to cobalt-60 teletherapy machines, linacs are complex and have higher training, infrastructure, and maintenance requirements, making them a less suitable option for radiotherapy in many LMICs.
With technical expert assistance from organizations such as the IAEA and others, many governments in African and other LMICs are building or expanding cancer therapy centers. Plans for acquisition and commissioning of equipment for the centers are often inconsistent with the available infrastructure and resources and instead are driven by the government’s desire to obtain state-of-the-art equipment. As a result, the more sophisticated linacs are often chosen over the simpler cobalt-60 teletherapy machines. While keeping with the international community’s desire to phase out use of cobalt-60 teletherapy, frequent power outages have led to disruptions in linac operation in some African countries. In addition, the slow internal process for requesting technical and maintenance support for linacs, the lack of local capabilities to provide that support, and the unavailability of replacement parts add to delays in cancer therapy delivery. Based on the committee’s case studies, it is likely that at least for the foreseeable future, cobalt-60 teletherapy will play an important role in cancer therapy in LMICs.
Recommendation G: Efforts by the U.S. government and other national and international organizations to reduce use of high-activity radioactive sources globally and in low- and middle-income countries should be driven by examination of the local resources, infrastructure, and needs. In situations in which local resources and infrastructure cannot support alternatives, efforts should focus on enhancing radiological security for existing radioactive sources, assisting with building the infrastructure, and supporting research and development projects to adjust the technologies to operate effectively in resource-constrained environments, for example, when there is unreliable electricity supply.
LMICs have an increasing need for cancer therapy capabilities and capacity. International technical assistance needs to take a holistic approach in assisting LMICs to build radiotherapy capabilities and capacity that involves addressing specific health needs; supporting the training of medical personnel in the use of linac technologies; and providing for adequate technical resources including for repairing linac machines and improving reliability of electricity supply. In countries or medical centers where cobalt-60 teletherapy cannot be replaced by linac technologies, efforts should focus on providing assistance to improve safety and security of radioactive sources.
The committee also observes that there is a growing market opportunity in LMICs for manufacturers of alternative technologies that can work effectively without needing frequent repairs and relying solely on electricity from unstable grids. At least two large companies, manufacturers of medical devices for radiotherapy, have invested in research and development of devices that could address the infrastructure challenges in LMICs that often prevent normal operation of a linac. In the United States, smaller companies, with support from NNSA, also have invested in research and development of machines that could address the infrastructure challenges in LMICs.