5
Engineering Targeted and Accessible Medicine
Spending for health care in the United States is enormous (Figure 5-1) and has grown over the years, from 5 percent of gross domestic product (GDP) in 1960 to nearly 18 percent in 2019. The largest fractions of this total are for hospital care (31 percent) and physician and clinical services (20 percent). The costs of prescription drugs have skyrocketed as well, despite a decrease in retail drug prices, and represent a growing share of health care costs (e.g., 3.8 percent in 2018, compared with 5.7 percent in 2019; CMS, 2020; Martin et al., 2021). On the other hand, revenue from the biotechnology sector, including drugs and agricultural and industrial products, is a significant contributor to the national economy, accounting for more than 2 percent of GDP in 2012 (Carlson, 2016).
Therapeutic drug molecules are either small molecules (molecular weight [MW] below 1000 Da) synthesized chemically, or biologic molecules of any MW produced by biological (cell-based) methods. Drug development today includes both therapeutic classes, although small molecules now account for roughly 70 percent of U.S. Food and Drug Administration (FDA) drug approvals and about 60 percent of U.S. market share (Makurvet, 2021). This is the case in part because, for small molecules, the cost of synthesis is lower and the delivery of therapeutic material is simpler. Biologics have the advantages
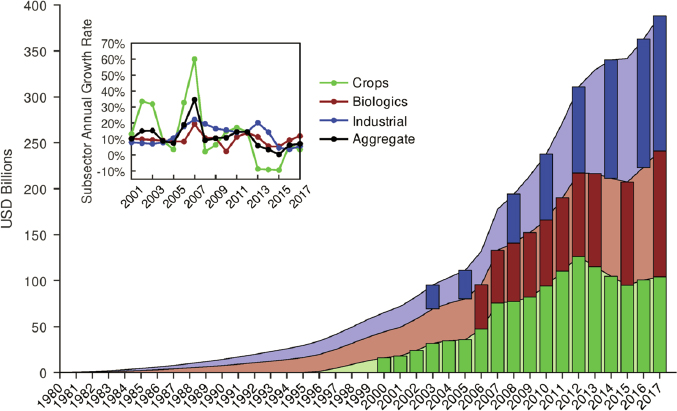
of offering highly selective targeting and, from an intellectual property perspective, being more difficult to copy. However, they can be much less stable; are unable to cross many biological barriers, including the blood–brain barrier; and are typically inactive when administered orally. Both small (chemical) and biologic molecules are likely to continue sharing the medical discovery and therapeutic markets, with the choice between the two depending on the application and disease at hand.
This chapter describes the role of chemical and biochemical engineering in human health applications, with a particular focus on biomolecular engineering. Following an overview of the role of biomolecular engineering in health and medicine, the chapter describes opportunities for chemical engineers to advance personalized medicine; improve therapeutics; understand the microbiome; design materials, devices, and delivery mechanisms; and develop hygiene technologies. Finally, the chapter examines how chemical engineers can contribute to addressing health disparities that result from societal inequity and reducing the costs of therapeutic treatments.
THE ROLE OF BIOMOLECULAR ENGINEERING IN HEALTH AND MEDICINE
Biomolecular engineers drive the development of computational and experimental techniques for identifying drug targets using multiscale modeling tools and data science approaches (see Chapter 8), and then employ organ-on-a-chip and/or tissue model development to replicate in vivo behavior. Small-molecule drug development and manufacturing still depend on chemical engineers who understand colloid science, particle dissolution, and multicomponent phase behavior to design formulations. For small-molecule
discovery, automated, high-throughput screening of potential drugs will continue to integrate chemical synthesis, modeling of binding surfaces, and automation. Scale-up and manufacturing of both small molecules and biologics also rely on traditional process development skills of biomolecular engineers in addition to novel approaches, such as the integration of production and purification.
The origins of biomolecular engineering as applied to therapeutics can be traced back centuries to the use of such organisms as baker’s yeast (Saccharomyces cerevisiae) to ferment grape juice and leaven bread. The first formal application of engineering principles to biological processes is often recognized as the production of penicillin in the 1930s and 1940s. The bacteriologist Sir Alexander Fleming is credited with discovering in 1928 the ability of an accidental mold growth to inhibit the growth of staphylococcus colonies, naming the active component of the mold penicillin. After significant work by a collaborative team of scientists and engineers to produce the drug at scale, this serendipitous discovery led to a major medical advance in the treatment of sepsis. Other similar compounds from molds led to the field of antibiotics, which of course remains critical in medicine to this day.
Despite the eventually profound impact of the discovery of penicillin, it was more than a decade later (in the late 1930s, as the world was poised to enter World War II) when Ernst Chain, Howard Florey, and Norman Heatley at Oxford identified the potential agent from Penicillium notatum (Florey, 1949). They worked to produce it economically by extracting it from the broth of the growing mold. Because of the intense bombings that began in the United Kingdom, Florey and Heatley visited the United States to try to address the needs for large-scale production; the collaborative transatlantic effort of biochemists, bacteriologists, physicians, and chemical engineers (and more) made it possible to produce penicillin biosynthetically, as chemical synthesis appeared to have little chance of success. Even biological routes were difficult—hundreds of scientists and engineers worked to create enough penicillin to treat all allied troops wounded in the D-Day invasion of Europe (~250,000 patients/month). Notably, Margaret Hutchinson Rousseau—the first woman to earn a PhD in chemical engineering and the first female member of the American Institute of Chemical Engineers (AIChE)—designed a deep-tank fermentation process for growing the surface-growing mold to enable penicillin production on a large scale. Among other significant advances were media development, strain improvement, and purification and recovery innovations that resulted in a 5,000-fold improvement in yields to 50 g/L (ACS and RSC, 1999; Gaynes, 2017). These efforts illustrate how classic chemical engineering concepts were applied to human health as early as the mid-20th century, ultimately contributing to the broader convergence of human health and engineering.
Since the first attempts to produce small molecules from biological organisms, the development of biologically derived products has increased. As described in Box 2-2 in Chapter 2, biochemical and biomolecular engineering and production of medicines have continued to grow, with major advances resulting from the development of recombinant DNA technology, the sequencing of genomes, the development of polymerase chain reaction (PCR), the discovery of induced pluripotent stem cells, and the discovery
and implementation of CRISPR (clustered regularly interspaced short palindromic repeats) (see Figure 5-2). The first recombinant protein therapeutic approved by the FDA was insulin (5.8 kDa) in 1982, derived via production in Escherichia coli (E. coli) with subsequent purification and refolding (Carter, 2011; Leader et al., 2008). Since the 1980s, many more proteins produced in E. coli have been approved, and they are still produced in this simple bacterium. However, mammalian cells, particularly Chinese hamster ovary (CHO) cells, have been used to produce around 70 percent of all recombinant therapeutics since the late 2010s (Jayapal et al., 2007; Wells and Robinson, 2017).
Today, monoclonal antibodies (mAbs) represent the highest volume of sales and a major focus of drug development. These therapeutics can bind to a specific antigen and have been developed as highly specific treatments for such diseases as cancer, asthma, arthritis, Crohn’s disease, migraines, and infectious diseases (Lu et al., 2020; Wells and Robinson, 2017). The first therapeutic mAb was muromonab-CD3, approved by the FDA in 1986, which was utilized in the treatment of transplant rejection until 2011. Humanizing antibodies, and later producing fully human mAbs as part of a library for protein engineering, rapidly accelerated the development of therapeutic antibodies (Jones et al., 1986; Tsurushita et al., 2005). Over the last 25 years, many antibodies have entered the market (Figure 5-3), with 61 mAbs being approved by the FDA for clinical use at the end of 2017 and an additional 18 new antibodies being approved from 2018 to 2019 (Lu et al., 2020).
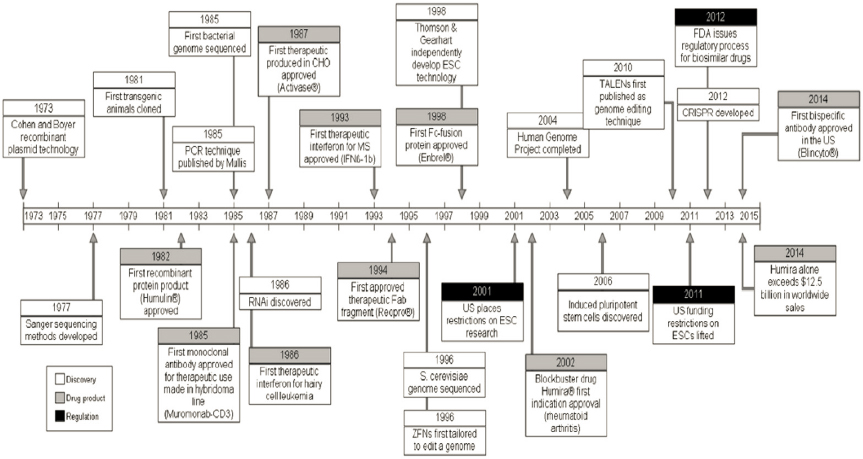
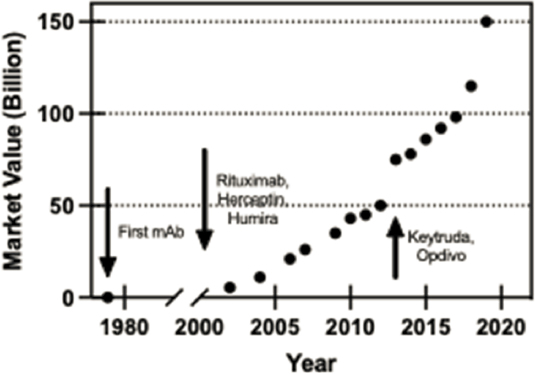
Modern biomolecular engineering is very much at the intersection of chemical engineering and molecular biology, biochemistry, materials science, and medicine. The role of chemical engineers in addressing problems in health and medicine continues to lie in process and scale-up, as well as in discovery and development of molecules and materials with pharmaceutical and medical applications. For example, the development of the small-molecule therapeutics Remdesivir and Molnupiravir highlights the value of biomolecular engineering in combatting the COVID-19 pandemic. However, the repercussions of the pandemic also serve as a reminder of the importance of the availability and transport of raw materials, as well as the critical importance of scale-up of manufacturing where medicines are needed and the fact that even in higher-income countries, health disparities can result in needless deaths.
The next 20 years of chemical and biomolecular engineering will feature opportunities in personalized medicine; advances in the engineering of biologic molecules, including proteins, nucleic acids, and such entities as viruses and cells; growth at the interface between materials and devices and health; the use of tools from systems and synthetic biology to understand biological networks and their intersections with data science and machine learning; development of the next steps in manufacturing; and the use of engineering approaches to address health equity and access to health care.
PERSONALIZED MEDICINE
An important area in which chemical engineering can contribute to the future of medicine is personalized medicine, which denotes the tailoring of treatments for individ-
ual health, including cell and gene therapies for patient-specific disorders, as well as preventive care regimens based on an individual’s genetic-disease propensity or biomarker presence. As more is understood about the role of genetics and environment in future disease, a continuing emphasis on personalized medicine is likely, with a clear role for chemical engineers in the development of appropriate models at scales ranging from atomic to systems for target discovery and the design of medicines based on those targets. The application of data science and modeling represents another opportunity for chemical engineers to contribute in this space.
Small-Molecule Manufacturing
For small molecules that serve as active pharmaceutical ingredients (APIs), a number of innovations could contribute to advances in applications during manufacturing. A detailed discussion of the importance of small molecules and related research opportunities for chemical engineers can be found in the recent report of the National Academies Innovations in Pharmaceutical Manufacturing on the Horizon: Technical Challenges, Regulatory Issues, and Recommendations (NASEM, 2021b). This section focuses on the application of additive manufacturing applied to personalized medicine. One such application is polypharmacy, which entails product formation through the use of 3-D printing. This method is of growing interest because in the United States, more than 40 percent of those over age 65 use five or more medicines, and 5 percent use more than ten (Kantor et al., 2015), creating complexity and confusion for patients. Polypharmacy is also common in Europe and Australia (Schöttker et al., 2017). Beyond the pharmaceutical implications of understanding how these medicines may interact in treating disease, the ability to deliver medicines appropriately in a safe and efficacious way and to ensure that the dosage and route of delivery are optimal is a need that chemical engineers and others can address through both systems biology and advances in manufacturing. In addition, the ability to combine multiple medicines into one delivery on a patient-demand basis would likely improve health care outcomes.
The use of 3-D manufacturing for APIs poses many technical challenges, including physics-based modeling of the fluid mechanics of drop formation and extrusion for liquid and powder solidification, as well as challenges involved in the extrusion process itself (NASEM, 2021b). Improving and simplifying the delivery of multiple drugs is a key opportunity for chemical engineers.
Additional opportunities for improvements in small-molecule manufacturing lie in predictive analytics and in the emerging field of translational medicine bioinformatics. Pharmaceutical companies use predictive analytics to develop algorithms that predict optimal conditions for robust production by leveraging large historical datasets. For low-dose or limited-duration drugs for which limited scale-up data are available, Bayesian statistics are used to estimate acceptable parameter ranges and product specifications. Translational medicine bioinformatics is an emerging field that draws on the fundamentals of chemical engineering and has the potential to transform personalized medicine. By analyzing raw data from DNA sequencing, patterns can be detected for specific mutations
or genes to help predict which small-molecule drugs a patient might respond to. This field holds the potential to turn biomarkers into clinical diagnoses.
An additional need is the implementation of plug-and-play analytics, allowing rapid shifts from one production process to another (e.g., for small-batch production), and more uniformity in data formats for analytical devices across vendors. As supply chains continue to be stretched, the widespread adoption and implementation of digital twinning, or simulated process models, to predict and plan for supply chain issues that affect downstream challenges (e.g., the availability of specific vials or packaging in a given number of months) will also be beneficial for biotechnology and pharmaceutical production.
Cell-Based Therapies
Another aspect of personalized medicine is cell-based therapies—most important, CAR (chimeric antigen receptor) T cell immunotherapy. Cancer cells typically carry a ligand that blocks a specific receptor (PD-1) on the cell surface of T lymphocytes, rendering this protective part of the human immune system ineffective, and thereby enabling cancer cells to evade the body’s natural defenses. CAR T cells are specially engineered to express CAR with a high affinity for tumor antigens. Immunotherapy—often termed immuno-oncology—has now become a mainstay of cancer treatment, with cell therapies representing the largest growth area and immunomodulation a close second. To some degree, the currently available therapies are influenced by what is known to be successful, with 22 cell and gene therapies being approved by the FDA in 2021 (FDA, 2021) and more than 460 targets in the current global pipeline (Xin Yu et al., 2019).
Therapeutic application of CAR T cells relies on retrieval of cells from a patient’s blood, isolation of these cells followed by engineering in vitro, and then reintroduction to the patient. This approach has been quite successful, particularly for leukemias; it is highly individualized, allowing high specificity. In 2017, Kymriah® became the first FDA-approved immunotherapy, wherein a patient’s blood is collected, and then individualized therapy is created by introducing a virus to the T cells to allow them to express a 4-1BB costimulatory domain to enhance cellular responses and express the CD-19 receptor. However, the cost of CAR T therapy is high for single-patient treatments, and the product’s stability and success can be patient specific. At present, a large number of immunotherapies are in clinical trials, and the annual growth rate is expected to be 15 percent for cell therapies and nearly 30 percent for gene therapies (Mullin, 2021).
New directions for improving reliability and lowering costs are an ongoing focus in the fields of biomolecular engineering and immunology. In particular, there is interest in scaling down manufacturing practices that were developed for large-scale culture to reduce the need for highly skilled workers (compared with the present process of collection of blood by clinical staff and its shipment to manufacturing sites). Also, to improve the reliability and efficacy of treatment, key variables or biomarkers that lead to successfully engineered cells need to be identified, and simple analysis methods developed (Wang et al., 2021b; Whitehead et al., 2020). The use of induced pluripotent stem cells has the potential to reduce patient-specific cell collection, but also requires improvements
in both modulating and measuring differentiation and manufacturing of these surface-dependent cells. Finally, T cell engineering requires viral vectors or particles that are manufactured with consistent quality and functionality.
Computational Tools and Modeling to Improve Personalized Medicine
Systems biology applied to physiology is an additional avenue for chemical engineers to contribute to personalized medicine. As early as the 1960s, Yeats and Urquhart (1962) noted that biological systems and their responses to physical stimuli can be understood using the engineering principles of process control. In fact, the impressive ability of biological systems to show resilience in the face of perturbations is possible with biological control systems that have components akin to their engineering counterparts, such as sensors, controllers, and actuators. Diseases can be seen as a malfunction or failure of a component in the control system, and quantitative approaches to understanding this behavior can benefit physicians’ ability to diagnose and treat these failures (Figure 5-4).
Chemical engineers have several opportunities to contribute to systems engineering of biological systems, such as sensor design and analysis, identification of fault detection (using physiological, cellular, metabolic, or other data to identify changes in function), and process modeling to represent complex relationships for biological systems and predict behavior (Ogunnaike, 2019). There are various approaches to developing these models (Janes et al., 2017); as they mature, the models will play a role in the health care industry in predicting and avoiding catastrophic health consequences for patients. Systems biology encompasses both the approach to framing questions about function and understanding of feedback, adaptation, and dynamics to enable the development of methods for treating or resolving illness (Janes et al., 2017). A key feature of systems biology is examination of the whole system, deconstruction or identification pathways and networks of biomolecules, and re-creation and integration of the information (Figure 5-5). As one might expect, the use of large, accessible data repositories (so-called big data) is a complementary approach to understanding biological behavior that can be used to shape information gained from the models (see Chapter 8).
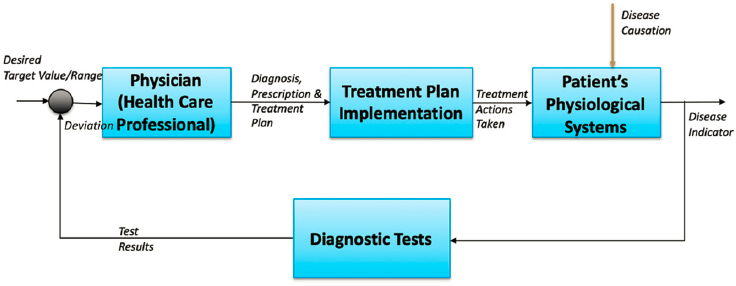
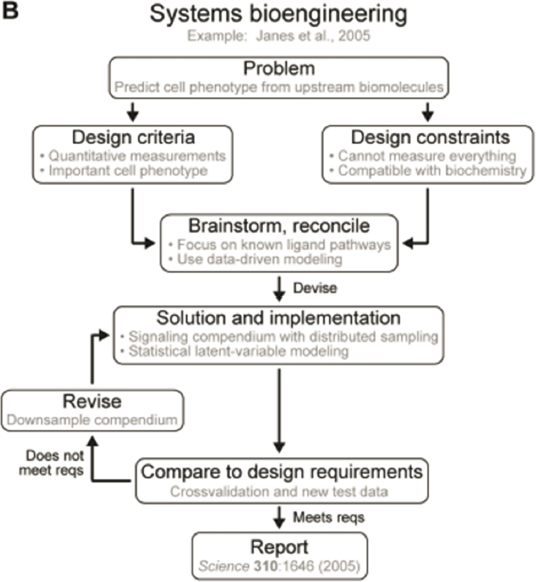
The continuous assessment of patient health—particularly for immunocompromised individuals—would improve understanding of the spread of disease and enable early-stage prevention and treatment. The potential ability to determine the status of a patient with autoimmune or other health conditions is highly attractive, yet this is currently a daunting task that requires a simple and well-designed method for identifying specific biomarkers, collecting leukocytes from the skin or blood, and evaluating the presence of inflammation or immune activation (Box 5-1). Other means of patient-state monitoring might include the design of nanoparticle or microparticle probes targeting specific cell types so they can be tracked and monitored over extended time periods.
ENGINEERING APPROACHES TO IMPROVING THERAPEUTICS
Vaccines as Biomolecular Therapeutic Agents
Although drug discovery is key to the development of new types of drugs, discovery is only the beginning of the development phase; significant challenges arise in the large-scale manufacture of drugs, including industrial-level product generation, purification, and formulation. The COVID-19 pandemic has highlighted some of the challenges
of bringing drugs to market. Key to the success of the rollout of COVID-19 vaccines, messenger RNA (mRNA) vaccines from Pfizer-BioNTech and Moderna, was the generation of production lines to accommodate large-scale production and formulation. Fortunately, Moderna had already gained experience in this area and built a manufacturing facility for the development of other vaccines that were approaching early clinical trials.
Protein-based biologic drugs have been manufactured using cell-based bioreactors to generate proteins for therapeutics, and a significant amount of biotechnology know-how is based on the engineering of bioreactors that require management of titers from microbes or mammalian cells, management of cell viability and oxygen and nutrient levels, and extensive removal of cellular debris and waste products as part of the purification process. Unlike these processes, the production of mRNA uses a cell-free enzymatic reaction; RNA polymerase is utilized in the presence of a DNA plasmid and an RNA promoter that accelerates amplification of the DNA sequence. Additional enzymatic
steps may also be used to modify the end groups of the mRNA. Because the process does not use cells, the concentration of the product can be much higher than is possible in a bioreactor for which cell density is limited and high concentrations of cells can lead to cell death and toxicity. And because the reactions are enzymatic, the reaction rates, yield, and purity are based on the nature of the promoter and the frequency of enzymatic errors that lead to short oligonucleotide impurities, double-stranded RNA, and similar by-products that need to be removed to create a pristine product free from potentially dangerous constituents. RNA self-replication machinery can be encoded in the sequence, thus increasing rates of generation of mRNA. Purification steps have involved high-performance liquid chromatography or other column-based chromatography. In general, the manufacture of mRNA therapeutics is a very new area, with a very different set of reagents, kinetics, products, and processes.
At the time that the SARS-CoV-2 virus appeared, Moderna had 10 other mRNA-based drugs approved by the FDA as Investigational New Drugs or for clinical trials, and had already launched a manufacturing facility for mRNA vaccines that were in various phases of clinical trial. Because the manufacturing machinery was already in place, the company was able to take full advantage of the highly versatile and modular nature of an mRNA vaccine, along with a significant funding stimulus from the U.S. government. Once the SARS-CoV-2 sequence had been revealed publicly, the U.S. National Institute of Allergy and Infectious Diseases collaborated with Moderna to determine a most probable target sequence from the spike protein, and Moderna generated DNA templates and launched production of a new mRNA encoding for the COVID-19 antigen within days, and using its platform, it took just 3 weeks to go from sequence to vaccine. By contrast, the turnaround time for a standard vaccine—typically derived from a known protein antigen or a deactivated form of the virus—can range from several months to years. The speed at which the mRNA vaccine was developed, tested in laboratory animals, and produced at levels sufficient for clinical trial was record breaking (Box 5-2).
Traditional vaccines use attenuated virus or virus capsids, but a great deal of work is currently being done on subunit vaccines—vaccines that rely on the use of a protein antigen that presents a key and broadly recognized epitope of the original infectious agent that is able to help initiate an immune response. The many advantages of this method include the ability to generate and mass produce a well-defined protein or polysaccharide biomolecule product instead of dealing with isolated viruses, typically using classic bioreactor synthesis; increased safety of the resulting vaccine for patients, including those who are immunocompromised; and the increased physical and thermal stability of the vaccine. Critical to the success of such vaccines is the ability to identify key sequences on the original infectious agent that can elicit the formation of neutralizing antibodies by B cells and/or initiate a T cell–mediated response. The nature of this response and its efficacy are highly dependent on the selection of the correct antigenic sequence or structure; ideally, this selected region would be a part of the virus that does not undergo many genetic mutations, so as to ensure efficacy against disease even in the presence of variants. Furthermore, subunit vaccines generally are not sufficiently immunogenic on their own
to induce a strong humoral or T cell response; therefore, for subunit vaccines that utilize much smaller portions of the protein antigen, adjuvants need to be added to enhance the body’s innate immune response and upregulate immune-cell activation. The future of vaccine development will include the use of genetic data mining and machine learning with biomolecular computation to enable a predictive approach to vaccine subunit selection, the ability to rapidly examine the immunogenic response of multiple protein subunits, and the establishment of protein scaffolds that facilitate such rapid assay approaches.
Chemical engineers will play a significant role in the development of the protein engineering and computational and biomolecular design space for the discovery of new antigens. The design of antigens, including the selection of specific sequences, and the use of different kinds of molecular adjuvants can affect the immunological response by directing different cellular pathways. For example, for certain viral infections, such as HIV, it is desirable to elicit a strong intracellular T cell response to prevent further viral replication; in other cases, a humoral response with highly effective neutralizing antibodies might be sought. Recent efforts have been focused on tissue-resident T cells and other memory T cells that can play critical roles in advancing immunity. The selection of the correct epitope to elicit a desired response and the engineering of the means of delivery of a vaccine to maximize that response introduce additional complexities that chemical engineering is well equipped to address. Aspects of vaccine delivery include the mode of delivery, control and impact of pharmacokinetics of antigen exposure, route of delivery, and degree and nature of uptake and presentation of antigens by antigen-presenting cells. Such materials as degradable polymers or other forms of delivery devices, such as microneedles for transdermal delivery, may be used to help control these factors or influence which cells are accessed upon release of the vaccine (Caudill et al., 2018; Prausnitz, 2017).
As important as the generation of antigenic molecules is, it is equally important to design their solubility and biocompatibility for ease of manufacture and downstream generation of purified vaccines. Because subunit vaccines are smaller biomolecular units, they also provide a more accessible route to creating manufacturing platforms that allow rapid vaccine development and scalable on-site manufacturing. An interesting biological engineering consideration is the selection of biological hosts for the manufacturing process. As an example, replacing mammalian CHO cells with rapidly dividing yeast cells for the production of new subunit vaccines can cut by a factor of four the time for both generation of clinical test compounds and, ultimately, manufacturing-scale generation (Brady and Love, 2021). The ability to use stable, easily transfected, and rapidly dividing cell lines can also enable the manufacture of key vaccines at low cost and high yield in underserved parts of the world (Matthews et al., 2017). These kinds of innovations are indicators of the potential impact of chemical engineers in enabling rapid response to diseases and a much more accessible and equitable approach to human health.
Engineered Proteins as Therapeutics
Engineered antibodies are now perhaps the largest and most expansive area of protein engineering. Since the first monoclonal antibody therapies were introduced in the
1980s, the domain of antibody therapies has exploded; by the end of December 2019, nearly 80 antibody-based therapies were on the market, and eight of the top ten drugs on the market were biologics (Lu et al., 2020). The design, development, and manufacturing of antibodies have been critical in the development of treatments of several disorders. Antibodies work via a highly specific binding and blocking mechanism that allows them to be highly effective inhibitors of biomolecules and specific cell-membrane receptors, and to bind to antigens to fight viral and other infectious agents.
Several challenges remain in the generation of antibodies on a commercial scale. Chief among them is the time and scale needed for antibody production. The cellular machinery needed to make complex antibody molecules is available in mammalian cells such as CHO; however, these kinds of cells typically exhibit low yields and slow rates of growth, and they can require significant maintenance because of their sensitivity to temperature and other environmental factors. Yeast and bacteria cells are much more productive, have rapid doubling times, and are readily manipulated to generate different protein sequences. Unfortunately, however, these systems need to produce proteins intracellularly, which requires cell lysis and complex separation steps. The design of the molecules can impact bioavailability, solubility during manufacture, and blood stability. Chemical engineers will play a key role in determining the best routes for antibody production.
The molecular design of antibodies is modular and provides a significant design space; the Fc (fragment crystallizable) determines the cell effector function—the specific cell type targeted and the nature of cellular impact. Recently, antibody fragments and engineered fusion proteins have also entered the market, utilizing variants or portions of the original antibody while introducing a range of advantages over full antibodies for a number of applications. Each of the Fab (fragment antigen-binding) components contains an inner binding region, and the single chain fragment (scFv) contains the recognition and binding sequence from the Fab. These forms allow for more direct incorporation of a much smaller binding molecule, thus providing molecules that do not require the complex manufacturing steps needed to produce whole antibodies. Protein engineering of these molecules has led to exciting therapeutic opportunities—the combination of Fab components from two different antibodies can yield bispecific antibodies with dual-binding capability, and it is also possible to fuse an antibody with a targeting protein to create a therapy that binds to specific cell types for targeted therapies. Furthermore, additional modifications can be made in the degree or type of glycosylation, the presentation of charge and charge heterogeneity, and control of the hydrophobic/hydrophilic nature of the molecule. These options represent myriad possibilities for therapies that have not yet been explored. Chemical engineers can manipulate these systems on the molecular level using rapid assay methods and computation in combination with studies of manufacturing properties to achieve high yields and lower overall cost.
Biomolecular engineering has had an enormous impact on therapeutic designs. It has come in a variety of modes, including computational peptide designs; display libraries of phage, yeast, and bacteria for target discovery; and protein engineering of antibodies and antibody fragments. The design of phage, bacteria, and yeast libraries has allowed the generation of numerous diverse peptide motifs to identify target binding agents (Arnold, 2018; Wittrup, 2001). Identification of specific ligands has been a key driver of biologics
discovery. Chemical engineers have made prominent contributions to this field through the introduction of quantitative analyses and optimization. These display tools have been extended to in vivo application, enabling direct in vivo identification of target-specific binding sequences for organs, which can enable targeted drug delivery—the so-called vascular zip code approach (Teesalu et al., 2012). Viral libraries have also been used to identify target-specific gene therapies. For example, adeno-associated virus libraries can be evolved to facilitate in vitro and in vivo gene delivery (Bartel et al., 2012). Such directed evolution approaches have contributed to efficient optimization of agents in the rather complex biological landscape.
Keeping proteins stable under physiological conditions for prolonged times is also a significant hurdle. Engineering of agents that promote protein stability continues to be an area of opportunity in chemical engineering. Biologics now represent a major class of therapeutics, monoclonal antibodies have emerged as the dominant therapeutic modality, and nucleic acid–based therapeutics are poised to increase their role in the therapeutic landscape. However, these delicate biologics require stringent storage conditions. Maintenance of a “cold chain” for frozen formulations represents a serious challenge in the future use of biologics, especially in resource-limited communities and geographic regions. Strategies for biologics stabilization have their foundation in protein biophysics, an area that has benefited from the active participation of chemical engineers. Fundamental studies founded in the thermodynamics of water structure, influenced heavily by chemical engineers, have laid the foundation for strategies for protein stabilization. Solutions for future challenges posed by the need for nucleic acid stability can potentially leverage the knowledge generated about protein stability.
MODELING AND UNDERSTANDING THE MICROBIOME
The complexity of the microbiome—which includes multiple cell types involved in both the microbial community of a given organ and the different host cells that support and respond to that community—presents a challenge for modeling of host–microbiome interactions to develop predictive solutions for human health (Box 5-3). The past 20 years have seen several advances in the toolsets available to chemical and biomolecular engineers for creating predictive models for cellular systems; these capabilities are enhanced when combined with access to large banks of genomic data. Genetic information about a microorganism, along with the location of key genes, can be determined, and this information, in combination with understanding of the biochemical and metabolic activities of the cell (effectively the reactome), makes it possible to determine the fully reconstructed signaling networks of cells. This information can then be converted to a mathematical representation and analysis so that computational approaches can be applied to enable predictive capabilities of specific network signaling pathways.
These genome-scale metabolic models (GEMs) have progressed and expanded in large part as a result of the constraints-based reconstruction and analysis (COBRA) approach (O’Brien et al., 2015). This approach narrows large numbers of possible pathways in optimizations by applying key constraints, such as nutrient availability and rate of uptake by cells. Experimental data can be used to enable cell-type and conditions-based
constraints that enable more specific models. More recent advances have made it possible for computational approaches to take into account dynamic genomics due to evolution, as well as changes in cell states. In the previous decade, the expression matrix (E-matrix) was introduced for computationally predicting the expression of genes in an organism through knowledge of its transcriptional and translational activity. Metabolic networks and E-matrices can now be used together for modeling of cellular membrane composition and thus of the impact of such environmental factors as reactive oxygen and hypoxic pH shifts (Chowdhury and Fong, 2020).
The advancement of these methods in metabolic engineering in general has opened up many opportunities to apply them to understanding the microbiome. With the toolsets provided by GEMs, it is possible to connect microbiome and host-cell interactions with final biological function (Chowdhury and Fong, 2020). These efforts are greatly facilitated by significant cross-institutional efforts, including the National Institutes of Health’s (NIH’s) Human Microbiome Project (HMP), a massive NIH Common Fund effort involving public and private institutions that led to identification and sequencing of the microorganisms in multiple human organs and the generation of tools for connecting
microorganism genomic data to disease state and function. A second phase of HMP—HMP2—led to a series of publications on the role of the gut microbiome in inflammatory bowel disease (IBD), preterm births, and diabetes (Nature, 2019). The gut microbiome is the most studied microbiome of the human body, and significant progress has been made toward building databases that will inform GEM models, as well as other computational approaches, particularly machine learning and artificial intelligence methods that require large datasets. An example of a comprehensive GEM model is AGORA (assembly of gut organisms through reconstruction and analysis; Magnusdottir et al., 2017), an approach that uses gut metabolic and genomic data to semiautomatically generate reconstructions of metabolic pathways for 773 independent gut microorganisms. This accomplishment is of great significance in illustrating the power of such models to assist in determining the functional behavior of myriad combinations of cells in consortia, thus enabling such capabilities as determination of predictors of disease identification of targets for treating disorders. In 2020, AGORA2 was introduced (Heinken et al., 2020). The number of microbes included increased 10-fold; additional factors, such as microbial drug degradation, were introduced; and both singular and pairwise bacteria members are considered. These kinds of models can lead to better understanding of disease (e.g., understanding the role of microbial consortia as opposed to individual bacteria in regulating key processes) and exhibit predictive capabilities (e.g., predicting patient susceptibility to IBD or Crohn’s disease based on nutritive deficiencies).
Cell Transfer Approaches to Medical Applications for the Microbiome
Increased understanding that the gut microbiome of healthy humans can be extremely beneficial for the management of infection, inflammation, and metabolism has led to the use of native human microbial consortia—the collections of different naturally occurring bacteria species that reside within the body—as a means of regulating disease (Colman and Rubin, 2014). The approach uses fecal matter transplants (FMTs) to transfer the healthy and established microbiome from donor to patient. The efficacy of this and related approaches, such as the use of oral pills formed from purified fecal matter, has been demonstrated to show efficacy in restoring the ability to fight gut-related infections (e.g., aggressive forms of colitis, severe sepsis, diarrhea) and are in clinical trials for many related conditions (Wischmeyer et al., 2016)—more than 900 clinical trials for these therapies were listed in clinicaltrials.gov in 2020 (Wang et al., 2021b). Chemical engineers can help advance these kinds of native microbiota-based therapies to a new level by addressing their expansion, cost, and scalability—for example, by investigating isolation and purification of native microbiota to enable more consistent therapeutic products and approaches that can be readily replicated. Much is to be gained as well from a deeper understanding of the impacts and effects of such therapies. Machine learning, in combination with information gained from genomics and systems-biology models, may enable more effective predictive methods and more personalized approaches to treating patients and pairing these therapies with given characterized FMT systems.
The use of native microbial consortia, or communities of microbes of different types and function, demonstrates the power of the synergism that exists among the many
different cells within the human microbiome. Cellular communities consist of independent cells that work together to share the labor of generating different metabolites, maintaining different roles in managing energy, producing molecules, and enabling breakdown or processing of different nutrients. The various bacterial components create supportive environments that enable the fully functional microbiome to provide dramatic gains in immunological and anti-infective properties. Together, these communities are more resistant to disease, and exhibit reduced metabolic burden and a broader range of function than individual microbe types. Therefore, the next step in microbiome therapeutic approaches is the engineering of microbes and their combination to form similarly synergized consortia with potentially greatly enhanced capabilities (McCarty and Ledesma-Amaro, 2019). Given the complexity of the interacting cellular systems and their various functions, a systems approach will be required to replicate important interdependencies while incorporating new or enhanced functionalities.
Synthetic Biology as a Tool for Engineering Microbes to Improve Microbiome Health
Bacteria have unique qualities that can be manipulated to enable the generation of synthetic microbiota with programmed function. In conjunction with gene editing tools, this work can yield a wide range of functions that depend on communication and interaction with other cell types. These functions include intercellular signaling, among them chemical, biophysical, and adhesion molecule–based interactions between cells, as well as ionic and electrical channels. Quorum-sensing capabilities in bacteria populations, based on the sensitivity of cells to an autoinducer molecule that increases concentration as cell density increases, are an example of a microbial behavior system that can be manipulated to advantage in synthetic communities. It is possible to engineer quorum-sensing mechanisms that are independent and thus completely orthogonal to native commensal bacteria, thus enabling unique or independent “programs” instituted on the basis of specific cell types that will be responsive to a given quorum-sensing signal. Other mechanisms of cell–cell communications include N-acyl-L-homoserine lactones (NHL) and autoinducing peptides that can cause bacterial-cell circuits, programmed into given microbial species, to turn on or off based on their concentrations (Hwang et al., 2018). These tools can be combined with additional means of triggering response; for example, bacteria can be designed to respond to exogenous signals. As another example, inducer molecules can be used to trigger the expression of transgenes that activate a promoter. Synthetic biology enables the design of independent gene regulatory systems, and this orthogonality makes it possible to generate a range of independent controls that can be used to manage complex systems with multiple organisms.
Synthetic biology tools such as CRISPR and the assembly of DNA parts or “circuits” that can control genetic expression on demand can be used to modify a microorganism by introducing the ability to generate a given molecular signal, gene regulator, or indicator in the presence of a given disease condition. Examples of singular commensal or symbiotic bacteria that have been engineered include
- E. coli that can generate glycotransferases that yield molecular inhibitors to dangerous enterotoxins that cause diarrhea,
- lactobacillus engineered to prevent infection through the generation of bacteria-killing lactoferrins,
- cholera-controlling microbes that express a regulator of virulence genes, and
- E. coli microbes engineered to secrete antimicrobial peptides that can reduce the effects of Salmonella (Tan et al., 2020b).
As shown in Figure 5-6, harmless commensal bacteria can be modified synthetically and then reintroduced to the microbiome in the gut using oral delivery or transplant approaches. These newly introduced bacteria are then able to incorporate into the native microbiota and generate molecules that can address infection or greatly lower the impact of toxins released by undesired bacterial infections.
Along with modifying susceptibilities to infection, microbes can be engineered to release regulators of the immune system. This ability is particularly relevant for the treatment of inflammatory disorders such as IBD; Lactis bacteria (a probiotic used in production of cheese and yogurt) can be modified to produce anti-inflammatory cytokines such as IL-10 to treat IBD effectively. This and related approaches have generated great interest in the use of engineered microbes for therapeutic applications for conditions ranging from infection and inflammation to metabolic disorders and potentially even endocrine and autoimmune disorders, such as diabetes and obesity (Tan et al., 2020b). Given recent findings regarding the gut microbiome–neurological connection and the likely advances in biological understanding of both the gut and other human microbiome communities with respect to disease and overall human health, this area of technology is likely to expand significantly in the next decade.
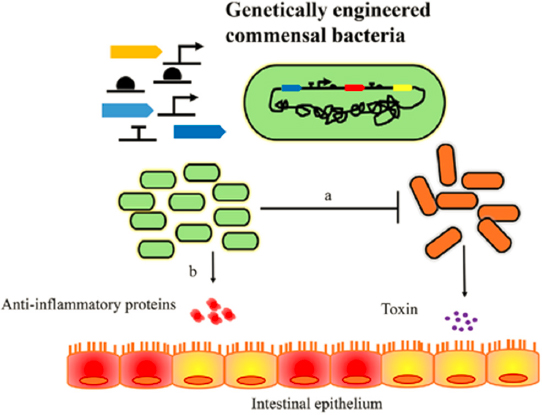
Activating molecules can include toxins, antibiotics, or specifically determined inducer molecules. Syntrophic dependencies, in which a molecule produced by one microorganism synergizes the activity or metabolism of another, further increase the complexity of these systems and enable the kinds of metabolic interdependencies found in nature (Harimoto and Danino, 2019; Hwang et al., 2018). It is clear that using these kinds of engineering tools to better design therapies based on engineered consortia will require applying understanding of complex signaling behaviors, modeling, and the manipulation of synthetic biology tools.
Chemical engineering, which has had a traditional focus on metabolic engineering, is a discipline particularly well poised to enable advances in these areas. In the late 20th century, the field of biochemical engineering brought forth the concept of the cell as a reactor and the use of cellular pathways to create molecules of interest with a high and controlled yield. Early developments in this field led to work on metabolic manipulation of cells to direct and control certain cellular reaction pathways over others. The microbiome is in some sense a highly tuned and sensitive bioreactor or series of bioreactors within the human body. The challenges for chemical engineering lie in designing cells for manipulating not only the generation of desired compounds but also the rate of generation, the selectivity for a given target, and the longer-term stability of the reacting system. Chemical engineers have embraced synthetic biology as a critical tool in addressing such problems and are well equipped to advance this work.
The unique skillsets of chemical engineers can contribute to gaining knowledge, enabling discovery, addressing disease, and enhancing human health through understanding and regulation of the human microbiome. Key challenges and opportunities include further advancement of synthetic biology tools that incorporate environmental and conditional responses, regulation of the reactome across multiple species, and engineering of cellular consortia to achieve patient-specific outcomes. The expansion of engineered therapies to other microbiomes will result in the need to determine effective modes of delivery to specific organs, which may require the engineering of new biomaterials that support the transfer of microbiota to different parts of the body. Along with advances in GEM methodologies, additional data science and computational approaches will likely become more important in predicting and regulating metabolism. Increased availability of data on the gut, skin, vaginal, oral, and other organ microbiomes will enable the use of artificial intelligence and machine learning algorithms, with anticipated increased use of models and computation to direct the early detection of potential disease and the application of preventive health measures to avoid disease.
DESIGN OF MATERIALS, DEVICES, AND DELIVERY MECHANISMS
Devices play an important role in the landscape of biomedical technologies. Devices that control drug administration are of special interest to chemical engineers and an area in which they have made significant strides. Such devices include external infusion pumps, implantable pumps, self-regulated delivery devices, and transdermal patches, among others (Anselmo and Mitragotri, 2014; Vargason et al., 2021). Implantable pumps
offer a patient-compliant means for long-term disease management and have had a transformative impact on diabetes, pain management, and neurological disorders.
Patients with type 1 diabetes are on lifelong treatment with insulin, and the need for frequent insulin injections represents a significant limitation in diabetes management. Implantable pumps offer an excellent alternative, especially for the delivery of basal insulin (Cescon et al., 2021; Shi et al., 2019; Wolkowicz et al., 2021). Several challenges, including those related to insulin stability and device biocompatibility, had to be overcome for these devices to reach the clinic. Another major challenge has been managing the compatibility of implanted pumps with the human body (Kleiner et al., 2014; Park and Park, 1996). Foreign devices, including implanted pumps, evoke a foreign-body response that includes the arrival of immune cells, leading ultimately to the formation of fibrous capsules, whose properties play an important role in determining the durability of the device. Chemical engineers have made important contributions to this field through understanding of the foreign-body response and the development of coatings to minimize fibrous capsule formation.
Control strategies are especially relevant for insulin pumps, for which an active control of insulin release is necessary to maintain euglycemia. A healthy pancreas adopts a complex control algorithm to control insulin release such that glucose levels remain within a tight window. Accordingly, chemical engineers have led extensive efforts to develop model-based and adaptive control strategies that enable communication between glucose sensors and insulin pumps. As glucose sensors have advanced to the point of providing continuous measurement over several days in patients, effective control algorithms have supported the development of closed-loop insulin delivery devices—the so-called artificial pancreas (Doyle et al., 2014). The paradigm of self-regulated pumps can be extended to other indications for which continuous and regulated delivery is essential. The development of such devices requires novel formulation strategies, pump designs, control algorithms, and continuous sensors, challenges for which chemical engineers are well suited.
Another exciting frontier for chemical engineering is the development of devices for completely noninvasive methods of drug delivery. Such devices can be placed on the skin, where they can offer a needle-free method of continuous and regulated drug administration. These transdermal patches provide a natural means for the sustained release of drugs (Prausnitz and Langer, 2008), providing the key benefit of active patient control and ease of termination when needed. Several transdermal patches are currently available, allowing easy delivery of nicotine and fentanyl, for example. However, these patches are limited to the delivery of small-molecule drugs because of the transport limitations of human skin, leaving many emerging drugs beyond their scope.
Several advances have been made in improving drug delivery across the skin to expand the use of transdermal patches to biologics. One such advance, and an area in which chemical engineers have already made an impact (Hao et al., 2017; Prausnitz, 2017), is microneedles, which penetrate the skin to a depth sufficient for delivering drugs but not for inducing pain. Microneedles are showing high potential, particularly for delivering vaccines, skin being an excellent organ for vaccine delivery because of the presence of immune cells. Further, microneedles carry vaccines in solid form, thus improving their
storage stability. Such solid, stable, self-administered vaccines could be particularly suitable for use during the current COVID-19 pandemic. Strategies have also been developed for enhancing noninvasive delivery of biologics through oral or inhalation routes, with enabling contributions from chemical engineers (Brown et al., 2020; Matthews et al., 2020; Morishita and Peppas, 2006).
The human body is a connected system of various organs, each designed for a unique function. While the macroscopic behavior of each organ has historically been studied in medicine, quantitative understanding and control of transport properties in these systems are limited. In the absence of this control, access to many organs is severely limited. At a fundamental level, transport is limited by the body’s natural metabolic processes and transport barriers. These biological barriers, while serving the important purpose of regulating the body’s metabolic functions, also limit how drugs can be delivered in the body (Figure 5-7). Accordingly, many potential drugs fail to reach their destination in the body, and thus most molecules never become drugs. This limitation ultimately reflects the high cost and lengthy timeframes of drug development.
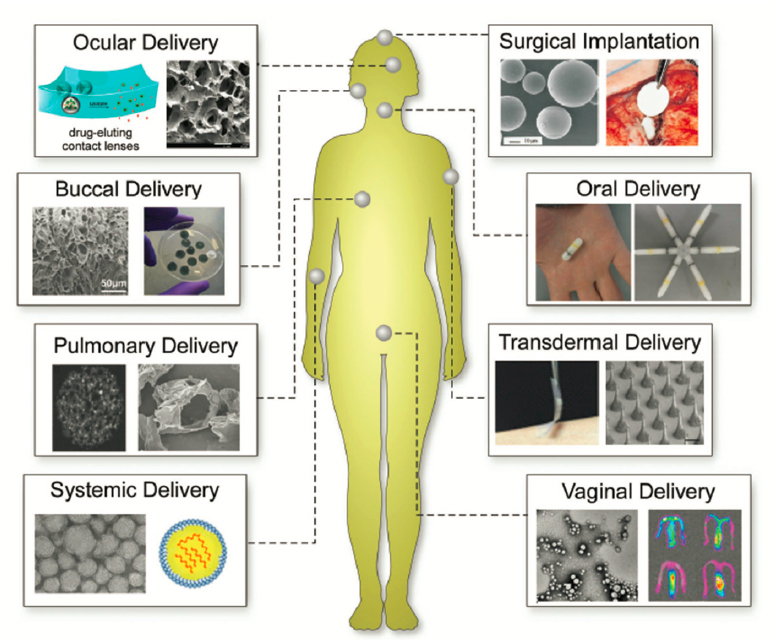
The early contributions of chemical engineers to small-molecule drug delivery were focused on developing encapsulation strategies to control the release of drugs. Macroscopic and microscopic depots have been designed to encapsulate small and large drugs. The most commonly used microsphere-based drug delivery systems consist of a suspension of polymer microspheres that can be delivered by subcutaneous injections. Fundamental studies describing the diffusion and degradation kinetics and mechanisms of diffusion in the polymer matrix, led by chemical engineers, have played an important role in the establishment of these systems (Ritger and Peppas, 1987). Triggered release of drugs from these polymeric depots, mediated by such external triggers as light, ultrasound, and magnetic fields, has allowed better control over the drug release (Sun et al., 2020). Chemical engineers have numerous opportunities to advance sustained-release depots, especially with respect to extending the release duration, which can further improve patient compliance. Such advances are especially relevant for such applications as ocular delivery, given the strong motivation to minimize the number of injections in the eye. Long-term compatibility of depot systems with the immune system also represents a continued challenge.
At a nanoscale level, the same polymeric systems have provided benefit for targeted drug delivery, a concept first introduced for the delivery of chemotherapeutic agents. Numerous efforts have been made to improve the targeting ability of nanoparticles by virtue of their surface modification of polyethylene glycol (PEG), which reduces surface protein adsorption and subsequent hepatic sequestration. Nanoparticles can be further modified by targeting ligands, including peptides, aptamers, and antibodies, to improve target accumulation (biomaterials are discussed in more detail in Chapter 7). A number of nanoparticles, including liposomes, polymeric nanoparticles, and inorganic nanoparticles, are already commercially available, and several are available in the clinic (Karabasz et al., 2020). Lipid nanoparticles have played a central role in packaging of mRNA to enable its stabilization and intracellular delivery (Mitchell et al., 2021). While a large number of materials, targeting moieties, and design strategies have been explored, achieving exquisite targeting remains an unmet need.
Moving forward, opportunities for chemical engineers in the field of drug delivery include developing a better understanding of transport processes and leveraging this understanding to accomplish better targeting. One of the key challenges is the limited spatial and temporal resolution of drug imaging in the body. Model systems (e.g., organs-on-chips) can provide a deeper understanding of such transport processes (Bhatia and Ingber, 2014; Ghaemmaghami et al., 2012). Drug delivery methods also need to be patient-centric; that is, they need to improve patient compliance, including such considerations as cost, simplicity, and convenience.
Building Sensors and Diagnostic Tools
Rapid, frequent, and inexpensive diagnosis is the foundation of successful health care. Blood-based diagnostic methods, including those in the routine medical checkup and
in focused disease diagnosis, have been the cornerstone of past and even current diagnostic infrastructure. With perhaps the notable exception of glucose monitoring, blood-based diagnosis is typically done in the hospital setting, requiring clinical supervision.
Extensive efforts have recently been made to shift away from this inconvenient, discrete operation of blood sampling to a new paradigm focused on continuous measurements of physiological markers. Most such advances have been made in glucose monitoring for diabetes (Lipani et al., 2018). Continuous, wearable devices now available for patient use measure glucose for days through a single wearable device. In addition to providing multiple measurements, continuous sensors offer an educational tool enabling patients to understand how their glucose levels respond to various metabolic and external factors (e.g., Brown et al., 2019). Efforts have also been made to measure glucose concentrations in a completely noninvasive manner, making use of spectroscopic methods, noninvasive collection of tissue fluid through the skin, or the use of sweat-based sensors. The latter devices measure various analytes present in the sweat and use these measurements to derive a physiological assessment (Chung et al., 2019). A variety of analytes are present in sweat, including small molecules, such as glucose and hormones, and large molecules, such as proteins. Improvements to sensitivity, accuracy, and modeling based on these measurements are needed if these devices are to achieve broad health care application.
In addition to molecule-based diagnosis, wearable sensors can perform a variety of other diagnostic measurements, including measurements of temperature, blood oxygenation, pulse, and even biopsy alternatives (Gao et al., 2019; Kim et al., 2019). The ability to derive these key measurements through a wearable sensor has already transformed the collection of human physiology data. Traditionally, these measurements were possible only using large, bulky sensors, often connected to stationary electronic processors and displays. However, the ability to perform these measurements in a truly nonintrusive manner has enabled the collection of information about human responses in real-life situations.
Extrapolating these trends into the future, wearable sensors are expected to collect massive amounts of information about human behavior in healthy and diseased conditions. Some of the technical challenges in this field include the development of sensors to accurately collect and measure the small amounts of analytes that are available in sweat. Another challenge lies in accurately correlating these measurements with blood concentrations, a challenge that pertains specifically to the transport of analytes from the blood into the interstitial fluid, and relating this information to individual patient health. Can models be developed using these data to predict catastrophic physiological events through such analysis? Can drug reactions be better understood or predicted through analysis of such massive amounts of data? Opportunities exist for chemical engineers to develop large-scale network models with which to understand the dynamics and connectivity of adverse events. By virtue of their training in understanding and appreciating multiscale dynamics, chemical engineers are especially well suited to undertaking this challenge. Personalization of drug therapies is an exciting opportunity to reduce adverse events without compromising therapeutic efficacy. However, data need to be available to support
such personalization at the design stage and reduction of adverse events at the follow-up stage.
Cell-, Organ-, Organism-on-a-Chip to Model Biological Functions
Drug development is typically a lengthy and expensive process, with 10–15 years and approximately $2.6 billion dollars typically being required for development of a new drug (DiMasi et al., 2016). A large fraction of this time and expense is associated with late preclinical and clinical development. Acceleration of lead identification enabled by high-throughput screening, rational drug design, or computational platforms has greatly enhanced the availability of early lead candidates. However, the transition of these leads to preclinical and clinical programs is limited by challenges associated with the unknowns involved in evaluating the interactions of the leads in the body. In fact, the overall probability that a drug entering clinical trials will be approved by the FDA is about 12 percent (DiMasi et al., 2016). Currently, despite advances in computational systems biology and in vitro models, more than 60 percent of these failures are due to lack of efficacy and another 30 percent to toxicity (Waring et al., 2015).
Another key limitation is the limited relevance of animal to human pathophysiology. Small animals, especially mice, are commonly used in preclinical studies, their use being driven largely by the existence of disease models in these rodent systems. Specifically, several genetically engineered murine models exist with which to assess some of the key biological aspects of a drug, including phenotypic disease manifestation, target specificity, and improved survival. However, translation of these benefits to the engineering aspects of drug development is severely limited. For example, some of the major biological barriers to drug transport in the body, such as skin and mucous membranes, are substantially different in mice and humans. Some key aspects of these barriers that dictate diffusion differ greatly between mice and humans, in some cases primarily because of the differences in body mass and in others because of the innate differences between these species. Beyond mice, the universe of large-animal models is highly fragmented, and their use depends on the disease in question. Ultimately, the nonhuman primate model, which is often a key preclinical model, is ethically, logistically, and financially challenging. In addition to limiting the speed of drug development, these hurdles bias the landscape of drug developers because the required resources and time are often a luxury available only to large companies. Tools to minimize the burden of preclinical drug development will not only reduce the cost and time of development and provide preclinical information that is more relevant to clinical programs, but also level the playing field for drug developers.
Several advances have been and continue to be achieved in the development of scaled-down microphysiological systems (the so-called organ-on-a-chip or human-body-on-a-chip) that will provide an output at least as predictive as animal testing (Low et al., 2021). This field was pioneered by the cosmetic industry with the goal of eliminating animal testing for its products. That strategic decision led to the development of in vitro human epidermis models that can provide meaningful information about the safety of cosmetic products (Faller et al., 2002). The last two decades have seen similar devices able to mimic the function of other vital organs, including the liver, brain, and lungs,
among others (Low et al., 2021). Such systems require considerations not only of the biology—that is, incorporation of different cells (e.g., lung epithelial cells, astrocytes, hepatocytes) in the system—but also of the engineering aspects of flow and interfaces. For example, the liver is the most well-perfused organ in the body, and its function depends on that. Hence, a liver-on-a-chip would need to account for the intricacies of vascular flow (Schepers et al., 2016). Such devices can play a role in assessing drug toxicity. Hepatotoxicity is a key safety concern for many drugs, and a means of screening for it at an earlier stage could substantially accelerate drug development. Liver chips could also help screen nanomedicines, which are actively cleared by the liver after their administration to the body. Similarly, a lung-on-a-chip would need to incorporate the key features of an air–water interface in the presence of lung surfactant and mucus. Such lung chips could aid in assessing the interactions of drugs with the lung microenvironment, a topic that has become critically important during the current COVID-19 pandemic (Saygili et al., 2021).
Systems that capture the key elements of the immune system in vitro are also expected to support the design of future therapeutic products. The immune system is central to many major health concerns, including infections, cancer, and autoimmune diseases. The immune system functions through extensive orchestration of many cell types, including dendritic cells, T cells, and others, to stimulate a holistic response to drugs and vaccines. Systems that capture the key events of such orchestration in vitro (e.g., lymphatics-on-a-chip) would provide insights into the interaction of therapeutics with the immune system.
Moving forward, such organs-on-a-chip will require active incorporation of biological and engineering aspects into the design (see Figure 5-8). From the biological perspective, incorporating all essential cell types in the system is critical. More is not necessarily better because the model systems need to capture the essential complexity of the organ in the human body while being as simple as possible. Critical as well will be maintaining the cells in a correct phenotypic state, and ensuring cellular communication in these systems is also critical. Such key attributes include the barrier function—for example, diffusive properties of tissues and permeation across tight junctions or dynamics arising from vascular flow. Such systems need to leverage advances in microfabrication to capture key structural complexities in organs and advances in biological tools, such as gene editing, to control cells, and they require the means to incorporate and address complexity in a tissue microenvironment.
From an engineering perspective, most nonorganoid-tissue chips have very low throughput. Thus only a few replicates can be performed at any one time, which limits the ability to screen thousands of potential hits during drug discovery. More automated and miniaturized systems will be needed for commercial use. In addition, most systems are fabricated in-house in academic laboratories, so reliability and reproducibility become limiting. Clear approaches to quality control are needed, including physiological validation (sensitivity, specificity, and precision). Particular opportunities of interest to chemical engineers include understanding the connectivity of various organs-on-chips and assessing the role of flow in cells and organs. Both development and validation of these systems are research areas that chemical engineers are well suited to address.
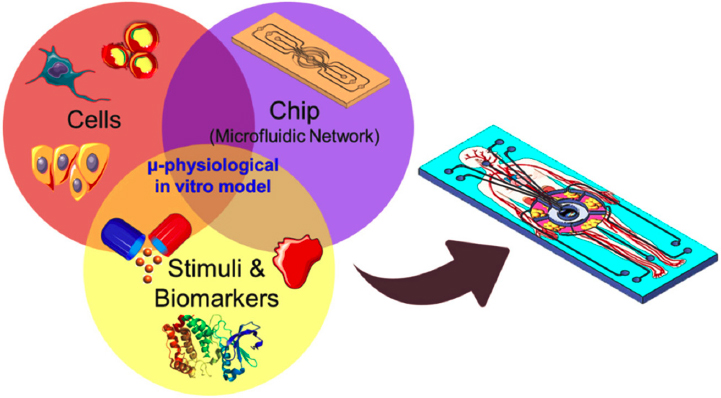
HYGIENE AND THE ROLE OF CHEMICAL ENGINEERING
Historically, engineers have played a major role in the development of hygiene and the sanitary infrastructure, developments that have led to an increase in life expectancy in much of the world. Indeed, diarrhea (endemic cholera) is no longer among the leading causes of death in world statistics (see Figure 4-8 in Chapter 4). Today in the era of COVID-19, chemical engineers, especially those collaborating with other scientists and engineers in environmental sciences and technology, have opportunities to contribute to societal health and well-being and help narrow the disparities between low- and middle income countries and higher-income countries.
Recent years have seen great strides in better understanding the link between indoor air quality and health,1 an area of growing concern in which chemical engineers can be expected to make major contributions. Within months of the onset of the COVID-19 pandemic, for example, long-lived aerosols (airborne particulates or droplets) were identified as the primary source of human-to-human transmission of the virus (Edwards et al., 2021; Prather et al., 2020) and a focal point for mitigation strategies, including the use of social distancing, face masks, and high-efficiency air filters. Figure 5-9 depicts the mechanistic framework for such superspreading events as the choir practice of the Skagit Valley Chorale, showing an aerosol size distribution from Bazant and Bush (2021). From their fundamental understanding of fluid mechanics, chemical engineers can readily confirm the time scale (many hours) for airborne suspension of aerosols in the 1-micron- to submicron-length scales in the figure, which indeed is the time scale described in the early COVID-19 literature (Prather et al., 2020).
___________________
1 The National Academies’ Committee on Emerging Science on Indoor Chemistry is currently examining the state of science regarding chemicals in indoor air.
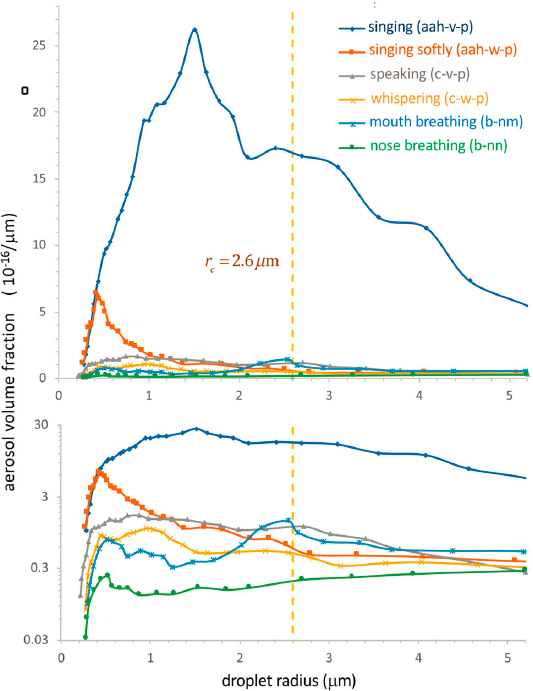
Chemical engineering and aerosol experts Edwards and colleagues (2021) took the analysis of aerosol size distribution in another useful direction—variation within the population as a function of several factors, including COVID-19 infection, age, and body mass index. They showed that all three of these factors can influence aerosol sizes by three orders of magnitude, and thus serve as a useful starting point for estimating of spreading distances and infection transmission rates. Ultimately, such insights on transmission rates can help guide policies (Samet et al., 2021) and mitigation efforts, such as the use of masks and air filters.
Hand sanitizers and related consumer/cleanser products are another aspect of hygiene that has risen to prominence during the COVID-19 pandemic, and an area in which chemical engineering has an important role to play, especially with respect to the balance
between antimicrobial/antiviral efficacy and product safety and environmental impact.2 The unprecedented high volume of use of hand sanitizers to combat COVID-19 has raised concerns regarding potential toxicity and/or negative impact on the environment (Mahmood et al., 2020) and rekindled interest in the development of new materials and formulations that can address these concerns.
Historically, the chemical engineer’s understanding of particulate and aerosol filtration has been based on mathematical models for capture by a single fiber (Spielman, 1977), with additional enhancements to build in geometric considerations, such as tortuosity and porosity. Such modeling approaches are common to other areas of chemical engineering that also analyze flow through porous media (e.g., modeling of oil reservoirs and mass transport in porous catalysts). Recent advances in coating materials, additive manufacturing, and systems engineering (Bezek et al., 2021; Christopherson et al., 2020) highlight further opportunities for filtration technologies for face masks and indoor filters. As attention moves past the current pandemic, and other potential challenges to indoor air quality and possible perturbants are better understood, chemical engineering will have growing opportunities to help develop materials that can change color or otherwise indicate health challenges so they can be addressed by physical barriers or treatment.
ENGINEERING SOLUTIONS FOR ACCESSIBILITY AND EQUITY IN HEALTH CARE
NIH defines health disparities as differences among specific population groups in the attainment of full health potential that can be measured by differences in incidence, prevalence, mortality, burden of disease, and other adverse health indicators. While the term “disparities” is often used or interpreted to reflect differences among racial or ethnic groups, disparities can exist across many other dimensions as well, such as gender, sexual orientation, age, disability status, socioeconomic status, and geographic location (NASEM, 2017). Despite overall improvements in population health over time, many such disparities have persisted, and in some cases, widened.
There is a persistent lack of awareness of and engagement with health disparities within science and engineering in both research and educational activities (Vazquez, 2018); indeed, one barrier to the greater involvement of engineers in health disparities research is their lack of knowledge in this area (McCullough and Williams, 2018). Although many health disparities result from systemic issues that can be addressed only through larger social changes, chemical engineers can still play a role in helping to resolve these issues. If they are to do so, however, these issues need to be introduced in the classroom and the workplace, imparting an understanding of the complexities and implications of health disparities, including the associated public health concerns and the social context of differential medical treatment based on race, gender, sexual orientation, age, disability
___________________
2 One aspect of this balance can be seen on the FDA webpage “FDA updates on hand sanitizers that consumers should not use,” with the following hazards (including product recalls): contains methanol or 1-propanol; contains microbial contamination; is subpotent, containing insufficient levels of ethyl alcohol, isopropyl alcohol, or benzalkonium chloride; is packaged in a container that resembles a food/beverage container, increasing risk of accidental ingestion.
status, socioeconomic status, and geographic location (Barabino, 2021). Addressing these disparities in chemical engineering education can even help attract students from diverse backgrounds (the importance of which is discussed in Chapter 9), students who are more likely to engage in exploring these issues as they go forward in the field (Thoman et al., 2015).
One opportunity for applying engineering solutions to reducing disparities is in low-resource settings. With respect to COVID-19, for example, chemical engineers can play a role in developing affordable vaccines for low-income populations who may not have access to traditional vaccine supply chains, distribution facilities, or cold-storage options (e.g., Frueh, 2020). Generally, creating appropriate technologies for low-resource settings requires that engineers consider not only the scientific rigor and effectiveness of the technologies but also their adaptability to local needs and ability to be maintained using resources the community can afford (TARSC, 2015). Today, while information is easily available to anyone with internet access, scientific tools and health care devices still prove to be expensive and inaccessible for many communities. As an example of addressing this problem, chemical engineers played a role in creating an appropriate and low-cost technology for diagnosing sickle cell disease in rural sub-Saharan Africa. What followed was a variety of new, low-cost, point-of-care testing devices that enable individuals to seek out sickle cell treatment in time (McGann and Hoppe, 2017; Nnodu et al., 2019; Oluwole et al., 2020).
Ethics, empathy, and attitudes are interrelated and important for eliminating disparities and building pathways to health equity. Conversations surrounding the ethics of engineering typically emphasize the integrity of the procedural steps of the scientific process. When creating and implementing new technologies, chemical engineers need to incorporate a broader set of ethical considerations and cultural competencies. Essential ethical considerations include the impact of new technologies or processes on low-resource communities and marginalized populations who experience greater health disparities, including how new treatments or technologies will be received among different cultures and populations and the impact on the environment. Chemical engineers have an opportunity to help reduce health disparities when they explicitly incorporate human-centered design into technologies to make them more accessible, equitable, and culturally sensitive. Collaboration across disciplines—engineers joining with clinicians, social scientists, policy makers, and members of the communities being served—will help address multilevel determinants of disparities and lead to better and more equitable interventions.
Another aspect of increasing accessibility to reduce disparities is lowering the cost of therapeutic interventions. The U.S. demand for monoclonal antibodies (mAbs) and a number of other biological compounds continues to grow, in part because of the increasing average age of people in the United States (Figure 5-10) and the diseases associated with aging, including cancer and cardiovascular and respiratory diseases. The success of mAbs in addressing those diseases drives demand. Unfortunately, the cost of producing biologics and the subsequent costs to consumers create pressure to improve flexibility and reduce costs so as to increase health care equity while maintaining reliability and stability during manufacturing and distribution.
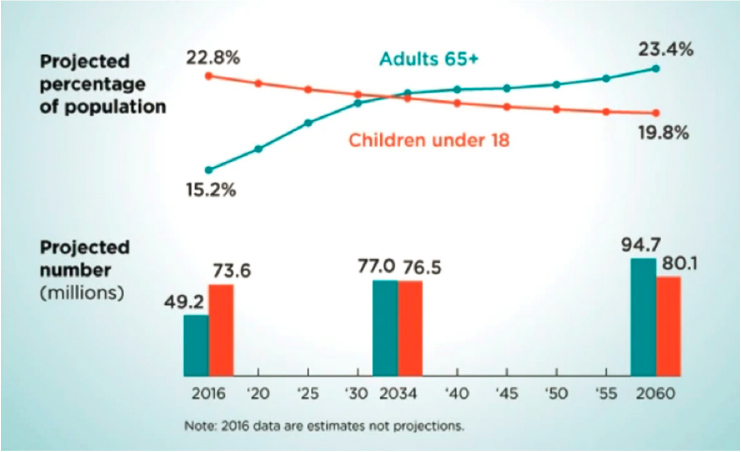
Approaches to reducing costs include streamlining workflows and increasing plant size, as well as developing strategies for producing higher protein yields per unit volume both in bioreactors and during purification and storage. One of the most obvious ways to produce high protein yields is to use continuous bioreactors, or perfusion culture. As in a traditional continuous reactor, this approach entails keeping catalyst (in this case the cells) within the reactor while adding fresh reactants (media) and removing product and spent media. Industry has long avoided this approach, as product yields have reached 2–10 g/L in fed-batch culture through media and process-based optimization. However, increased application of single-use technologies has opened up the possibility of broader application of perfusion technology, which can lead to three-fold increases in volumetric productivity (Bausch et al., 2019). In addition, expanding manufacturing space quickly is much more challenging with traditional plant designs, with new facilities costing more than $400 million and taking 5 years to build (Jagschies, 2020).
Some avenues toward improving perfusion culture processes include better scaled-down models to enable early development and screening of cells and optimization of media, which during traditional industrial development can take 3–6 months. Blending of concentrated media stocks by in-line dilution is also needed; even four-fold concentrates can save time and resources. Needed as well is the ability to combine these blending and perfusion runs with adaptive control technology driven by models of nutrient consumption, as well as improved cell-line development to ensure that the cells provide robust product (yield and quality) over the length of time expected for growth (2–3 months). In addition, supply chain issues, such as quality and consistency of raw material, become even more important with a perfusion approach to minimize product variance. Regulatory issues become more significant as well in that the product may change composition with
time (Allison et al., 2015); thus, improved control technology and defined product batches could lead to better acceptance of this approach.
One of the most important directions for reducing costs is moving beyond traditional model organisms and cells, particularly mammalian cells used for the production of therapeutic antibodies. Alternative cells to meet the catalytic needs of metabolic engineering for greener chemistries, wastewater treatment, and biomass conversion are the focus of other chapters of this report (Chapters 3, 4, and 6, respectively). For therapeutic applications, cell-free systems may provide opportunities to address on-demand therapeutics and orphan disease treatments (e.g., Swartz, 2012). As an alternative to mammalian cells, microbial hosts provide the advantages of reduced production costs and shorter process times in both development and production.
For antibodies, the addition of complex sugars, or glycosylation, particularly of a type that addresses efficacy and lack of immunogenicity, has limited the broader applicability of nonmammalian systems. Alternative cell types need to allow rapid growth; authentic protein processing and secretion; and the ability to engineer the cell readily, including with the use of genomic tools; these cell types also need to lack mammalian viral infectivity (Matthews et al., 2017). A number of chemical engineers have already made compelling cases for and shown successes in developing and implementing genetic tools for the use of yeasts, from Pichia pastoris to K. lactis, Y. lipolytica, and K. phaffi (Hamilton et al., 2006; Jiang et al., 2019; Miller and Alper, 2019; Panuwatsuk and Da Silva, 2003); S. cervisiae and P. pastoris are already FDA-approved production organisms for vaccines and cytokines. To enable widespread application, continued improvements in volumetric productivities are needed, as are improved genetic tools and models for metabolism, as well as a better understanding of why some products are not well expressed.
More broadly, developing technologies or tools that replicate the capabilities of their expensive counterparts—so-called frugal science—enables out-of-the-box solutions for global science and health care. For example, considering the different parts of key devices and creating alternative low-cost parts resulted in the creation of a $1 hearing aid (Sinha et al., 2020) and a hand-powered paper centrifuge (<$0.25) that enables blood separation in resource-poor settings (Bhamla et al., 2017).
CHALLENGES AND OPPORTUNITIES
Modern biomolecular engineering is very much at the intersection of chemical engineering and molecular biology, biochemistry, materials science, and medicine. Current challenges for applications in health and medicine include advancing personalized medicine and the engineering of biological molecules, including proteins, nucleic acids, and other entities such as viruses and cells; bridging the interface between materials and devices and health; improving the use of tools from systems and synthetic biology to understand biological networks and the intersections with data science and machine learning; developing the next steps in manufacturing; and using engineering approaches to address equity and access to health care. All of these challenges present opportunities for chemical engineers to apply systems-level approaches and their ability to work across
disciplines. Specific opportunities include the application of systems engineering to biological systems in such areas as
- sensor design and analysis;
- identification of fault detection (using physiological, cellular, metabolic, or other data to identify changes to function);
- process modeling to represent complex relationships for biological systems and predict behavior; and
- understanding and modification of molecular pathways and genomic networks involved in the regulation of normal physiology, as well as disease.
Opportunities to apply quantitative chemical engineering skills to immunology include cancer immunotherapies, vaccine design, and therapeutic treatments for infectious diseases and autoimmune disorders. The development of completely noninvasive methods for drug delivery represents an exciting frontier of device- and materials-based strategies. Chemical engineers are also well positioned to advance work on sustained-release depots and targeted delivery of therapeutics.
The demand for mAbs, therapeutic proteins, and mRNA therapeutics continues to grow, in part because of the increasing average age of people in the United States. Unfortunately, the costs to produce biologics and the subsequent costs to the consumer create pressure to improve flexibility, reduce costs, and increase health care equity while maintaining reliability and stability during manufacturing and distribution. This challenge provides an opportunity for chemical engineers to develop novel bioprocess and cell-based improvements through collaborations with biologists and biochemists.
Across all these areas, interdisciplinary and cross-sector collaborations will be critical, as will coordination across the federal agencies with responsibilities in these areas. An additional major challenge for chemical engineers is their lack of awareness of health disparities. Introducing these issues in the classroom will enable future chemical engineers to play an active role in reducing health inequities. Furthermore, increased diversity of both students and instructors in the classroom will provide a broader perspective on the challenges requiring engineering solutions.
Recommendation 5-1: Federal research investments in biomolecular engineering should be directed to fundamental research to
- advance personalized medicine and the engineering of biological molecules, including proteins, nucleic acids, and other entities such as viruses and cells;
- bridge the interface between materials and devices and health;
- improve the use of tools from systems and synthetic biology to understand biological networks and the intersections with data science and computational approaches; and
- develop engineering approaches to reduce costs and improve equity and access to health care.
Recommendation 5-2: Researchers in academic and government laboratories and industry practitioners should form interdisciplinary, cross-sector collaborations to develop pilot- and demonstration-scale projects in advanced pharmaceutical manufacturing processes.