7
Novel and Improved Materials for the 21st Century
Chemical engineers are deeply involved in the design, synthesis, processing, manufacturing, and ultimately disposal of materials of all kinds, and the connections between the fields of materials science and chemical engineering are numerous and diffuse. A taxonomy might suggest that materials scientists and engineers are concerned mainly with materials structure, properties, and performance, while a chemical engineer would likely focus less on performance and more on materials processing, but there are as many exceptions to that generalization as there are examples. In industry, the distinction is usually unmeasurable. Full coverage of the myriad dimensions of chemical engineering’s role in the materials economy would require its own report, but this chapter highlights several important aspects and key opportunities for the future. For example, materials research in academic chemical engineering departments will include work in polymer science, rheology, catalysis, biomaterials, nanomaterials, electronic materials, self-assembly, and soft matter; several of these subjects have been discussed in earlier, application-focused chapters of this report.
Chemical engineers have been responsible for many advances in materials design and development. An example is the reverse osmosis membrane for water desalination made of cellulose acetate and developed by chemical engineers in the 1950s (Cohen and Glater, 2010). Likewise, many of the polymeric materials used in regenerative engineering and drug delivery have emerged over decades from the laboratories of chemical engineers. Other contributions include new catalysts and zeolites (Ahmed, 2007), Gore-Tex®, and the automobile catalytic convertor.
Chemical engineers have played a key role in materials processing and system design that enable plants to make useful amounts of materials safely. They also are developing solutions for the consequences of decades of plastic generation, which has left the world awash in plastic waste. And they bring rigorous life-cycle assessment (LCA) to the processes that produce materials, as examined in greater detail in Chapter 6.
The economic and environmental burden of electronic waste rivals that of plastics—indeed, the fastest-growing segment of the global solid waste stream is electronics and electrical waste (Kaya, 2016)—and poses a challenge even more complicated to address. Consumer products such as mobile phones and personal computers (PCs) contain
more than 1,000 different chemical products, and more than 260 million PCs were sold in 2019 alone (Gartner, 2020). Gold, silver, copper, and palladium are the most valuable metals found in the waste stream, but they are challenging to recover. To gain perspective on the magnitude of the problem, consider that an economically viable gold mine yields 5 g of gold per ton of ore, while 1 ton of discarded cell phones can yield as much as 150 g of gold, 100 kg of copper, and 3 kg of silver (Nimpuno and Scruggs, 2011).
This chapter explores four areas of materials research, design, and production in which chemical engineers are particularly active: polymer science and engineering, complex fluids and soft matter, biomaterials, and electronic materials. These four areas are but a subset of materials work done by chemical engineers, and a deeper survey would yield its own report. Other areas that the committee did not explore include advanced (non–petroleum-based) performance fluids, such as lubricants or high-temperature heat transfer fluids; mixture formulation in general (beyond complex fluids); materials for separations beyond water purification (discussed in Chapter 4); and construction materials such as concrete and asphalt,1 an area that could lead to new collaborations with civil engineers. In addition, there may be a role for chemical engineering in quantum materials and quantum information technologies.2 Catalytic materials and applications in the energy transition are discussed in Chapter 3.
POLYMER SCIENCE AND ENGINEERING
In the 100 years since the publication of Hermann Staudinger’s landmark paper Über Polymerisation (1920)—which marked the beginning of an ability to design plastics with infinitely tunable properties, moldability/processability, and remarkably low expense—polymers have infiltrated every aspect of people’s lives. The purification of water, the preservation of food, the clothing people wear, the diapers on babies, the vehicles used for transport, the components of computers, and the delivery of medicines to the body all contribute to reliance on a global polymer industry whose products have grown in volume to more than 350 million metric tons per year since 1950 (Figure 7-1). Chemical engineers have been central to the development of this industry from its inception because of their integrated understanding of chemical synthesis and catalysis, thermodynamics, transport/rheology, and process/systems design. Indeed, the understanding of polymer rheology and design of processing equipment to handle highly viscous polymer melts represents a triumph of chemical engineering. Over this 100-year timeframe, the field of polymer science, including the contributions of chemical engineering, has become increasingly molecular in focus, leading to the ability to create new materials that integrate new polymer chemistries, topologies, and assemblies.
___________________
1 The National Academies’ Committee on Repurposing Plastics Waste in Infrastructure will explore the effectiveness and utility of plastic waste in asphalt mixes and other materials used in infrastructure, which may present opportunities for chemical engineers to collaborate with other disciplines.
2 The National Academies’ Committee on Identifying Opportunities at the Interface of Chemistry and Quantum Information Science is currently examining the research needed to make progress in this area.
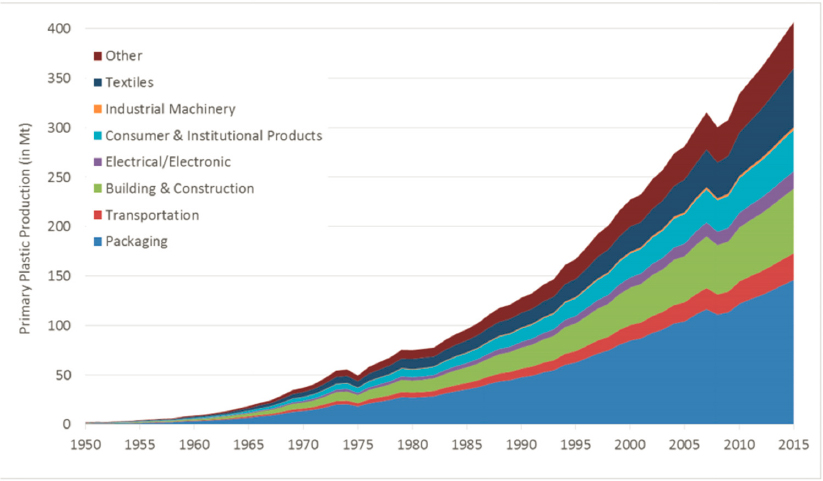
Chemical engineers’ backgrounds in polymer chemistry, thermodynamics, and kinetics have been especially well suited to designing and understanding the interplay between thermodynamic and kinetic driving forces acting through the atomic- (monomer), nanoscopic- (sequence, chain shape), and mesoscopic-length scales that lead to self-assembly—ultimately determining the macroscopic functional properties of a polymeric material. Block copolymers are one example of such hierarchically assembled materials. The ability to control the properties of the individual blocks to induce hierarchical order and control polymer macroscopic properties has enabled such applications as thermoplastic elastomers, semiconductors, nanolithographic masks and patterns, and ion-conducting solid-state electrolytes. Directed self-assembly of block copolymers led to the ability to make nanometer-scale patterns with high fidelity across macroscopic areas to rival stereolithography. New opportunities arise as one considers new assemblies in which the building blocks are more complex, are potentially dynamic, and change under external stimuli. In this way, both the molecular-scale structure and the macroscopic properties could adapt with time and situation to lead to truly active and responsive materials. Chemical engineers are well positioned to address the molecular engineering of hierarchical assembled polymers, which will rely on the design of chain conformation, mesoscopic structure, and macroscopic function in an integrated manner. For example, noncovalent interactions (e.g., metal-ligand, electrostatic, hydrophobic, hydrogen-bonding interactions) lead to dynamic, transient bonding with multiple handles to control multiple length and time scales simultaneously.
As laid out in the National Science Foundation workshop report Frontiers of Polymer Science and Engineering, making the next leap forward in the design of functional materials will require understanding, predicting, and utilizing molecular building blocks, sequence, conformation, and chirality to direct mesoscopic structure on desired time and energy scales to control macroscopic polymer properties (Bates et al., 2017). Further, while the ability to predict structure across length scales of six orders of magnitude has improved dramatically, it is now necessary to learn how to predict properties and behavior in order to guide material design. Within the next 20 years, the capability to design polymers from the bottom up for target applications will likely advance to a point at which the desired properties and behavior of a polymer can be specified and, using a combination of multiscale simulation and artificial intelligence (AI), the building blocks and the processing strategy can be designed to make it.
The ability to rationally embed information and function in synthetic macromolecules by controlling monomer sequence opens the door to polymers with the sophistication of biological molecules (Figure 7-2) and potentially with the ability to meet “the grand challenge of precision control over polymer structure and function” (Bates et al., 2017). Recently, strategies for controlling monomer sequence have evolved to enable synthesis of sequence-controlled synthetic polymer chains at gram scale (Lutz et al., 2013), allowing for molecular weight, architecture, and chain end control through highly efficient coupling reactions and step-wise automated synthesis and purification. Two fundamental questions that need to be answered if the potential of these advances is to be realized: What monomeric sequence should be incorporated? and What level of control is necessary to yield new structures and properties? (Bates et al., 2017). Now that almost anything can be made, what should be made? Even a single 80-mer chain composed of two different repeat units permits 6 × 1023 distinct sequences. In this Avogadro-scale design space, predictive tools that allow selection of specific targets of assembly become essential, as a design driven by intuition is no longer useful.
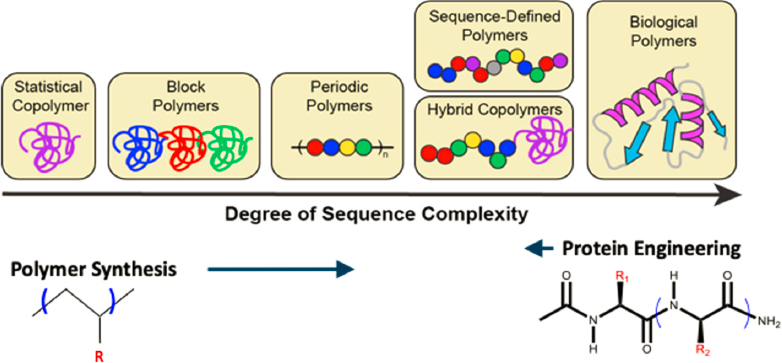
While the future of designed, molecularly engineered polymers-on-demand is exciting, responsible care of the environment demands a paradigm shift to include life-cycle considerations in the design imperatives. The combination of ultralow cost, scalability, and utility has led to the widespread use of plastics in every aspect of modern life. As a result, society has accumulated a huge debt of polymers that will never degrade. Global production of plastics has risen exponentially in the 70 years since their first mass production, from less than 2 Mt in 1950 to 359 Mt in 2018 (PlasticsEurope, 2019), and production is projected to double again in the decade ahead (Jambeck et al., 2015). Plastics are found in industries as diverse as food, medical devices, electronics, transportation, and construction. Packaging is one of the most common applications for plastics, and most packages are used once and then discarded after a life cycle of much less than 1 year (Teuten et al., 2009).
Mismanagement of plastic waste has contributed to persistent, visible environmental pollution (Rahimi and García, 2017), the formation and widespread dispersal of microplastic fragments, and leaching of contaminants that impact the health of ecosystems (UNEP, 2021). Only about 9 percent of plastic waste is recycled, and almost all is converted via mechanical recycling to lower-value materials (downcycling; Geyer et al., 2017). The combination of widespread use of plastics and the failure to deal with their end-of-life phase has brought the plastic-waste problem to the forefront of the world’s attention. The extreme aspect ratio and entanglement of polymer molecules give plastics the unique properties that led to their widespread use over the last century, but these same features—combined with the breadth of designer chemistries (and designer properties) of commodity plastics—present significant challenges to the recycling or upcycling of plastics to monomers or high-value products (see Chapter 6).
The development of feasible depolymerization processes requires fundamental understanding of catalysis, polymer chemistry, and the rapidly changing melt rheology during processing, and all of this knowledge is in the domain of chemical engineering. Similarly, chemical engineers are well poised to develop alternative, scalable plastics with properties equal to or better than those of current commodity plastics and with a greener life cycle.
COMPLEX FLUIDS AND SOFT MATTER
Traditionally, chemical engineers have designed and formulated functional fluids that are exploited in fields ranging from food, personal care, and pharmaceuticals to active braking fluids and bulletproof vests. These so-called complex fluids often include functional structures that form spontaneously by self-assembly or under external fields by directed assembly. The structures can represent equilibrium states, dynamical steady states, or kinetically trapped states. Such formulations have been developed to sequester sparingly soluble or delicate molecules, to modify rheology, and to control phase behavior (Larson, 1999). In the past two decades, chemical engineers have been at the forefront of developing scalable strategies for advanced functional material assembly in complex fluids and soft matter. The advent of nanotechnology lent urgency to this area, as the promise of advanced functionality relied on the development of efficient and scalable schemes for
incorporating microscopic and colloidal building blocks into larger structures (Box 7-1). Although this area of research is the modern version of colloid science and chemistry, its academic center in the United States now rests firmly in chemical engineering departments.
Surfactants
Surface active agents (surfactants) are found in nearly every household product and pharmaceutical formulation and are used industrially in processes ranging from emulsion polymerization to enhanced oil recovery. Historically, surfactants have been synthesized from petroleum feedstocks, although considerable progress has been made in the synthesis of surfactants from plant-based feedstocks. For example, nonionic surfactants made with sugar-based glucoside hydrophilic moieties instead of petroleum-sourced ethylene oxide groups have the advantage of coming from a renewable resource. In the same spirit, plant-based oils can be used to synthesize the hydrophobic portions of surfactant molecules. Catalytic conversion of biomass can create surfactant molecules with properties (e.g., tolerance in hard water) superior to those of conventional materials (Park et al., 2016).
Surfactants can be used at low concentrations to modify and control the surface tension and other properties of interfaces. Chemical engineers have made leading contributions to understanding of the statics and dynamics of far-from-equilibrium soft materials, including multiphase fluid systems that contain dispersed droplets or bubbles, surfactants, and other adsorbed materials. Examples include droplets in emulsions, bubbles in foams, and dispersions of one polymer in another (a polymer composite). These systems are typically dominated by complex dynamics at the interfaces. For example, surface-active molecules or materials self-assemble by adsorption on the surfaces of droplets and bubbles. These surfactant monolayers generate rich stress conditions that alter the effective stress of composite systems and can determine the stability of the dispersions to a dynamic perturbation. This perturbation can occur under processing or aging, and can determine the behavior of emulsions, foams, and composites commonly exploited in fields as diverse as personal care, pharmaceuticals, foods, and the design of tires.
The response of the system to a perturbation depends on the composition of the interface, which evolves over time. Understanding of the dynamics of surfactants and adsorbing species is built on insights from chemical engineering into mass transport and thermodynamics. This transport often occurs in the presence of flow and deformation of the interface and can depend strongly on flow conditions. Understanding of these stress conditions comes from chemical engineering’s long history of studying the fluid mechanics of multiphase flows with nonideal complex stresses (Scriven, 1960). Chemical engineers have addressed these issues by understanding droplet and bubble dynamics and have developed paradigms with which to understand the nonlinear dependence of interface mobility and surfactant concentration (Stebe et al., 1991) and to reveal the modes of droplet extension, deformation, and breakup (Stone and Leal, 1989). In extensional flows, drops break up to form tiny droplets with diameters far smaller than that of the parent drop (Taylor, 1934). Chemical engineers have revealed how the presence and distribution of surfactant dictate this occurrence (Anna et al., 2003; Eggleton et al., 2001; Pozrikidis, 1997). These are classic examples in which far-from-equilibrium effects occurring in self-assembled structures determine the dynamics, stability, and processing conditions of soft-matter systems.
The self- and directed assembly of materials on interfaces remains an exciting field. Interfaces are inherently open systems in contact with two bulk phases, and are sites for accumulation of (denatured) proteins, macromolecules, lipids, and particles, all of which can self-assemble, interact, form structures, and influence dispersion behavior. Chemical engineers continue to design interfacial probes to understand interface dynamics (Crocker et al., 2000), including those that exploit Brownian motion in the interface (Squires and Mason, 2009) and those driven externally (Reynaert et al., 2008). Chemical engineers design formulations for delivery to interfaces, with broad impacts that include the design of lung surfactant replacements for premature infants (Alonso et al., 2004) and of surfactant formulations for oil spills (Owoseni et al., 2014). Many of the concepts developed in these inquiries are now used to study materials assembly at complex fluid interfaces (Kaz et al., 2012) for functional materials and sensing applications (Sivakumar et al., 2009).
At higher concentrations in aqueous solutions, surfactant molecules self-assemble into a variety of micellar aggregates, and at even higher concentrations form various liquid crystal phases. The hydrophobic core of a micelle can be used to solubilize a hydrophobic molecule, and micellar solutions are the base of many formulations for water-insoluble drugs. Under some conditions, micelles can grow into long, flexible cylinders that entangle and give rise to highly viscous and viscoelastic solutions that are useful, for example, as fracking fluids for oil fields (Samuel et al., 2000).
The combination of a hydrophilic material (water) and a hydrophobic material (oil) with a surfactant in most cases creates an emulsion that is thermodynamically unstable. Appropriate mixing and shear, together with use of the right kind and concentration of surfactant, can produce emulsions that are long-lived or easily reemulsified by the consumer. Such emulsions, in which the dispersed drops are micron sized or larger, are common in consumer products and the food industry. Their structure may be water droplets in oil (W/O) or oil in water (O/W). It is possible as well to produce multiple emulsions, such as a water drop in an oil drop within another water drop (W/O/W). Additional processing can drive the droplet size smaller and create nanoemulsions, in which droplets are as small as 10 nm (Helgeson, 2016). Multiple nanoemulsions can also be made, and nanoemulsions are very attractive as drug delivery vehicles (Zhang et al., 2018a). Finally, under some circumstances, water, oil, and a surfactant can form a thermodynamically stable solution called a microemulsion, which contains microdomains of oil and water. It is an unfortunate nomenclature, but the characteristic domain size in a microemulsion is smaller than that of a nanoemulsion.
The formulation and optimization of complex fluids draws on core chemical engineering concepts from thermodynamics, transport, and kinetics while recognizing the key role of molecular forces. Chemical engineers are likely to continue to be at the forefront of this work.
Nanoparticles
Among the most exciting developments in soft matter in the last 20 years is the design, synthesis, and assembly of nanoparticles—colloidal particles ranging in size from
one to a few thousand nanometers. Just as whole new classes of polymer architectures can now be designed and synthesized through molecular engineering principles and made into complex functional materials, nanoparticles represent a new class of building block with tremendous potential for functional materials. The size of nanoparticles means they are controlled by the same laws of statistical thermodynamics that control polymers and complex fluids. Beyond the micron-sized polystyrene (PS), polymethylmethacrylate (PMMA), or silica colloidal particles long studied by chemical engineers, today’s nanoparticles can be made from a variety of materials (e.g., gold, silver, CdTe/S/Se, PbS/Se). Nanoparticles such as these are grown in solution, often as faceted nanocrystals with polyhedral shape. The size and shape of nanoparticles can be controlled using various techniques, yielding hundreds of different possible shapes with nearly monodisperse size distributions. Lithographic techniques now make possible essentially any particle shape. By combining shape with anisotropic interparticle interactions, practically any nanoparticle building block is possible. Spheroidal particles of PS or PMMA can be coated anisotropically with gold or platinum, or made of multiple materials, to form patchy particles called Janus particles. A Janus particle—where the two halves of the particle have different interactions with the solution—is the particle equivalent of a block copolymer. Particle analogs of triblock copolymers are also possible, as are particle analogs of star and other copolymers. These patchy particles are conferred valency by the surface pattern resulting from the synthesis and subsequent coating or functionalization (Glotzer and Solomon, 2007). This valency, combined with particle rigidity, gives rise to unexpected self-assembled structures, many of which are isostructural to atomic and molecular crystals but on larger length scale (Figure 7-3).
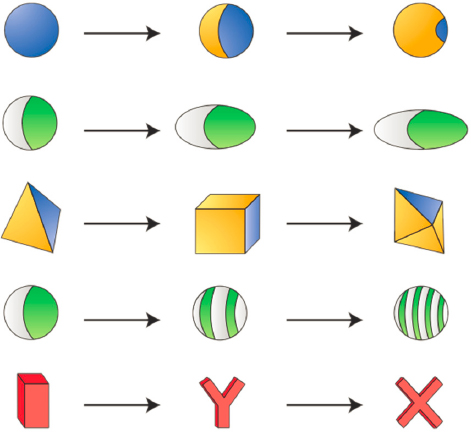
In the near future, it will be possible to make nanoparticles of any size and shape, out of almost any combination of atomic elements or in a core/shell structure, and functionalized with any type of surface ligands—including alkanes, dendrimers, DNA, and proteins—into any arbitrary surface pattern. These patchy particles are next-generation building blocks for self-assembly into complex and functional structures. For example, DNA-functionalized noble metal nanoparticles are programmable atom elements that provide a powerful path to a wide range of self-assembled colloidal crystal structures (Kim et al., 2006; Laramy et al., 2019; Tian et al., 2020b). Mixtures of particles functionalized with complementary DNA linkers can form colloidal cocrystals, in principle, of arbitrary complexity limited only by the shapes of the particles and human imagination. Even one type of particle with self-complementary linkers can, because of its anisotropic shape, self-assemble into complex structures, such as a colloidal clathrate crystal with more than 100 particles in its unit cell (Lin et al., 2017). The interplay between enthalpy and entropy and between thermodynamics and kinetics is central to assembly engineering of new materials from nanoparticles and falls squarely within the expertise of chemical engineering. The same question posed above for block copolymers—Now that we can make anything, what should we make?—applies here as well. Theory and computer simulation are essential for finding the “sweet spots” in the vast design spaces available for patchy particles. Data science, and in particular deep learning with neural networks, has an important role to play in nanoparticle design for self-assembly. Alchemical potentials (van Anders et al., 2015) and other methods (Sherman et al., 2020) will enable inverse design of nanoparticles for targeted applications.
Beyond the self-assembly of complex structures, the next decade will see increased effort to understand, design, and engineer the thermodynamics and kinetics of assembly processes. Engineering assembly processes, or “assembly engineering,” will make possible functional materials, reconfigurable materials, materials that rely on metastable structures, and soft metamaterials. Assembly engineering will also make possible materials inversely designed to have unique combinations of properties not typically found together, enabling wholly new classes of materials for a wide range of applications.
Assembly engineering may also involve directed assembly—for example, using external electromagnetic fields that dynamically rearrange suspended colloids to modify suspension rheology (as used in active brakes) and optics (as used in electronic paper e-ink). Sometimes, the field is one that comes from the complex fluid itself. The most obvious of such fields is surface tension; capillarity lies at the heart of some of the most widely adopted schemes for organizing colloidal building blocks. Nanoparticles are often spread and compressed on air–water interfaces of Langmuir troughs to form self-assembled structures trapped at the air–water interface. Nano- and microparticles are commonly deposited on solid surfaces using capillary assembly methods that rely on the collection and deposition of the building blocks near the three-phase contact line. So great are surface tension and the change in energy when particles adsorb that adsorbed particles can become trapped on the interface. Pickering emulsions are droplets stabilized by kinetically trapped monolayers of particles at their interfaces. Bijels are their bicontinuous counterparts, stabilized by jammed particle layers. The structures formed using surface tension
can be exploited directly, can be polymerized, or can serve as templates for other advanced materials. For example, polymerized bijels have been explored as hollow fiber membranes, and metallized bagels have been explored as high-surface-area electrodes.
Janus and other patchy particles can be made into “active” particles that are self-propelled. One approach is to coat half the particle with a material (e.g., palladium) so that the particle is propelled via catalytic activity when the material encounters a “fuel” (e.g., hydrogen peroxide). Another approach is to drive nanoparticles with external magnetic or electric fields (Han et al., 2018). Collections of active particles can produce complex emergent behavior far from equilibrium, including swarming and motility-induced phase separation, even in the absence of interparticle attractive interactions (Marchetti et al., 2013; Takatori and Brady, 2015). The field of active matter is a burgeoning one that, as it relies on nonequilibrium thermodynamics, falls naturally within the portfolio of chemical engineering research. By combining assembly engineering with active nanoparticles, chemical engineers are well positioned to create novel materials and material machines with robotic function at the nanoscale.
BIOMATERIALS
The design of biomaterials has seen strong growth in chemical engineering as a large number of advances have led to clinical translation that leverages degradable and biologically derived polymer systems. Materials design has had an influence in areas ranging from controlled localized release systems to small interfering RNA (siRNA) delivery, and a significant impact on the advancement of medicine and improvement in patient health. Key areas for advances in the biomaterials field include regenerative engineering, wound healing, systemic and localized delivery of nucleic acids, and the delivery to and detection and imaging of regions of the body that present unique barriers or opportunities.
The biomaterials field can also provide solutions in areas beyond human health. Applications with unique promise include areas that support plant and animal science, as well as the design of plant- or organic-based materials systems that could enable biologically derived or biomimetic polymers. This section focuses primarily on health-related applications, with a brief discussion of other areas in which biomaterials will be important. In addition the recent National Academies report Frontiers of Materials Research: A Decadal Survey (NASEM, 2019d) includes an extensive discussion of research opportunities in biomaterials.
Materials for Regenerative Engineering
The need for methods for generation or repair of tissues and organs is compelling. The ability to create materials systems that can be tuned to mimic cellular environments, including the extracellular matrix of tissues and organs, and the biological cues they present opens up the pathway to deriving organs and repairing tissues. Wound healing; the regeneration of bone and cartilage; the replacement of key organs, such as skin or liver; and the repair of tissues, such as cardiac tissue, are just some of the applications enabled
by the generation of materials matrices and components that can be combined to support appropriate living cell types. Key to the function of biomaterials for these applications is the ability to provide settings with the appropriate physical, chemical, and mechanical cues to enable single- or multicellular systems to arrange, order, and structure into organized tissues. The array of chemistries available for generating tissue scaffolds is vast; however, there are overarching requirements for low material toxicity, minimal immunogenicity, and the ability to match mechanical properties of the original organs or tissues. In many cases, moreover, the biomaterial needs to be capable of undergoing controlled degradation as native tissue is evolved to replace the synthetic material scaffold. The complexity of the biological tissues that require replication introduces additional constraints on the materials systems that can be used for these applications.
Polymeric materials, including a number of polymer systems that form hydrogels, have played a critical role in the design and development of biomaterials. These advances have been made possible by the range of synthetic backbones available; the ability to “design in” degradability based on hydrolytic susceptibility, pH, or the presence of protease-susceptible bonds; and the ease of functionalization of polymeric constructs—all of which make them excellent scaffolds for presenting a range of different proteins, peptides, and other molecular cues on their surfaces. Along with polymers, a range of mineral composite materials—including hydroxyapatite; porous silica; and other engineered materials, such as carbon nanomaterials and inorganic or hybrid materials systems—have provided biophysical cues, such as mechanical stiffness, and sources for biomineralization of hard tissues, such as bone.
Key advances include the development of dynamic synthetic hydrogels whose chemistry can be reversibly activated using light or physical or chemical stimuli to induce changes from one state to another. The more traditional chemically cross-linked hydrogel network is static, which prevents these networks from undergoing remodeling or supporting different tissue development or growth stages. However, more recent capabilities have allowed the incorporation of photoresponsive groups that enable 3-D patterning of surface functional groups. Such groups can act as cellular cues, develop reversible cross-linking to modify mechanical stiffness, or enable materials that can reversibly exhibit different mechanical states (Rosales and Anseth, 2016). The use of dynamic chemical bonds and secondary interactions could enable such changes in biological state as hypoxia, cell state, or the presence of certain signaling molecules to trigger changes in a way that enables cycling (Figure 7-4).
More recent efforts have also been directed toward engineering materials that can incorporate and support multicellular systems—essentially allowing for the generation of 2-D and, more particularly, 3-D cellular cocultures supported by materials that enable cell–cell interactions like those in biological systems. To a large extent, work has focused on direct replacement or replication of tissues or organs; however, there has also been an interest in engineered multicellular living systems (M-CELS; Kamm et al., 2018), which include in vitro cellular cultures that can exhibit additional, unique, or modified functions, such as on-demand production of a biochemical upon sensing of a trigger molecule, or actuation of cellular strips upon a given electrical or chemical stimulus. These concepts
require the design of materials systems that can provide not only physical support but also a matrix that will enable cell–cell configurations that best enable function.
Demands for multicellular systems, whether for synthetic organs or living machines, include the need to achieve larger and more complex materials systems that can support angiogenesis and the development of a vascular system that can provide oxygen and nutrients to cells while in culture. For these reasons, some of the key challenges in this area include supporting endothelial and smooth muscle cells while promoting the sprouting and growth of stable vessels; degradable materials systems more readily accommodate a growing vascular system. Organization of cells of different types is also required for sufficient function; biomaterials that can be patterned, aligned, or otherwise manipulated to organize cells selectively are of great interest for these applications.
Additive manufacturing, or 3-D printing, is an important tool for biomaterials synthesis. Biological inks, or bio-inks, which involve the integration of cells into biologically compatible materials using water-based solutions or suspensions, can be used with additive manufacturing methods to create complex patterned structures containing specific cells in precise arrangements, as with organs and functional tissues (Murphy and Atala, 2014). Because different tissues exhibit quite different mechanical and chemical properties, a range of different kinds of bio-inks are needed to address these various needs. Furthermore, needs for additional materials include ways to generate cellular suspensions in droplet form while protecting cells from extreme shear forces during printing. The materials used need to have sufficient cohesion to maintain shape and enable adhesion to the scaffold. Ultimately, the softer scaffolds typically used may also suffer from mechanical failure during assembly because of the weight of the printed scaffold, and this balance between mechanical support and cell compatibility sometimes leads to trade-offs (Decante et al., 2021). It is anticipated that the development of new and more readily manipulated materials systems for bio-inks that address these issues will be a focus of chemical and materials engineers.
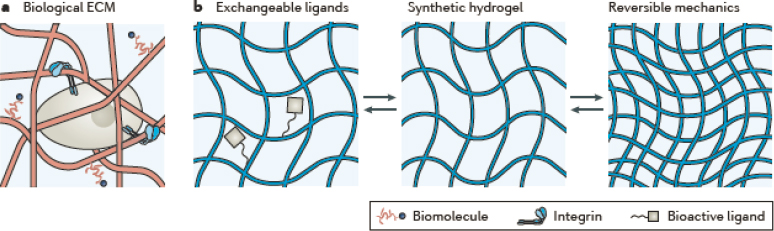
Additional needed developments include the ability to incorporate vasculature into these systems—a key issue requiring more than precision cellular placement. While cellular systems can be printed in minutes, it can take days for precursor or stem cells to develop appropriate stable vasculature. It is therefore difficult to incorporate blood vessels directly into such tissues, and patterning of endothelial cells is insufficient for creating functional systems. Finally, a greater ability to mimic the native extracellular matrix environment is necessary to achieve systems that can develop and evolve to generate integrated organs. Although it is possible to imbed growth factors and other proteins into existing matrices, it would be preferable to pattern them by printing at higher resolutions (Ng et al., 2019).
It is important to note the importance of the above techniques not only for tissue regeneration but also for organ-on-chip applications, which replicate organ function using microfluidic devices. Such applications are discussed in Chapter 5.
Materials for Drug Delivery
A second large and significant area of growth for biomaterials is the generation of materials systems that can deliver drugs to the body with control over the timing of release; the release location; and the targeting of specific tissues, cells, or organs (see also Chapter 5). The past few decades have led to a detailed understanding of the chemistries of biodegradable materials capable of achieving desired time-release profiles and of the thermodynamics that control self-assembled materials, such as block copolymers and liposomes. A range of accomplishments in both more traditional and new materials systems enable drugs to be encapsulated effectively and to retain their efficacy while decreasing undesirable side effects and cellular or organ-level toxicity.
A large array of functional polymers, lipids, silica, and inorganic hybrid systems have been developed that enable different modes of preprogrammed delivery or delivery on demand in response to specific stimuli. Recent developments have led to therapies that involve the administration of biologic molecules, ranging from proteins and peptides to nucleic acids, such as siRNA and messenger RNA (mRNA). Although many advances have been achieved in the field of controlled drug delivery, several key challenges and opportunities remain following the discovery of new therapeutic approaches and the introduction of new experimental and computational tools to aid in the understanding of these materials.
Systemic Delivery and Nanomedicine
The development of nanoparticle systems—which include polymeric and liposomal nanoparticles, as well as inorganic and hybrid nanomaterials—offers another opportunity for chemical engineers to make an impact in biomaterials. Applications of nanoparticles are promising, particularly for cancer treatment, for which nanoparticles are believed to have an advantage in sequestering toxic drugs from the rest of the body and targeting tumors. Unfortunately, although safety and efficacy have improved and cancer
nanomedicines are currently in clinical trials, the delivery of nanoparticles in patients is complex and presents several challenges.
The surface chemistry, shape, size, and mechanical properties of nanomaterials affect their circulation half-life within the blood stream, their ability to access tumors by crossing the endothelial barriers that make up the vascular wall, and their downstream release characteristics as they progress from circulation to accumulation in the tissue of the target tumor. Nanomaterials can be designed to present a range of different surface ligands, including synthetic and native biomolecules, peptides, and proteins, such as antibodies that exhibit strong affinities for surface-bound proteins or other biomarkers on the surfaces of target cells, such as tumor cells. With more detailed investigations of the impact of physical and chemical features and nanoparticle composition, the field is recognizing that these characteristics can yield significant differences in nanoparticle transport and distribution in the body. This increased understanding will guide how nanocarriers are deployed against a targeted disease such as cancer.
Nascent opportunities in the cancer field include nanoparticles that can contain combination therapies addressing two or more vulnerabilities found to be synergistic in each tumor type. Of particular importance is the ability not only to encapsulate dual therapies in a singular particle system, which can present unique materials challenges, but also to control when these therapeutics are released, as in some cases, synergy depends on the timing and staging of release. An advantage of nanoparticle systems is the ability to tune these release profiles and ensure that target cells receive a sufficient dose of each drug.
Nanomedicine beyond Cancer
Although cancer is by far the disease setting most studied in nanomedicine, nanocarriers have also been used in several other biomedical applications. Nanoparticle systems show potential for the targeted delivery of antibiotic or antiviral therapeutics to infected regions of the body (Yeh et al., 2020), particularly in the case of inflamed and infected regions such as lung or cardiac tissue. Additionally, nanoparticles can be designed to “home” to immune cells in circulation or in the lymph nodes based on nanoparticle ligands designed to bind to cellular surface markers. In this case, nanoparticles are fundamentally interesting for the delivery of vaccines. Several organs present unique challenges for delivery that have been addressed using nanomedicine. For example, nanoparticles with dense, highly hydrated polyethylene glycol (PEG) brushes have been developed to penetrate the unique barrier of corneal tissue for eye treatments, leading to commercial products for addressing macular degeneration. As another example, because nanoparticles are naturally filtered through the liver, they also prove to be useful systems for delivery of drugs to the liver. As nanomaterials are found to be highly versatile and broad in material properties for adapting to challenging organs, their use is anticipated in several additional areas, including avascular cartilage tissues and the ultimately challenging blood–brain barrier, an area that will be extremely impactful for the delivery of treatments for neurological disorders.
High-Throughput and Data Science Approaches to Discovery
A more systematic approach to nanoparticle design can ensure that the field advances in a manner that contributes meaningfully to patient care. High-throughput methods have been devised for generating large numbers of nanoparticles with differing characteristics (e.g., Dahlman et al., 2017). When such approaches are coupled with the ability to perform large-scale in vitro screens, it is possible to discern nanocarrier candidates that exhibit increased efficacy for delivery. The potential to generate significant databases that include interactions of nanocarriers with a range of tumor-associated cells, including patient-derived cells, can also provide the basis for machine learning approaches for understanding and guiding the design of nanocarriers that target tumor or tumor-associated cells, such as stromal or immune cells. These studies need to be coupled with in vivo studies to understand the ultimate transport and trafficking properties and targeting efficacy; such studies may also be designed in a high-throughput fashion using DNA barcoding or chemical barcoding methods to label nanoparticles, in conjunction with state-of-the-art imaging and detection methods coupled with appropriate cell-sorting algorithms. It is also possible to consider these methods as a means of determining possible biomarkers for patients whose tumors readily take up nanoparticles or whose tumor vasculature may exhibit the enhanced permeation and retention effect, thus making it possible to identify candidates who will benefit most from nanoparticle therapies. These approaches represent a renaissance in understanding of and much more rapid evolution of nanoparticle design. Future work will extend recent accomplishments to a much broader set of nanocarrier compositions and structures, allowing a greater amount of nanomaterials discovery toward tailored nanoparticle function.
Nucleic Acid Delivery
Perhaps the most important and impactful recent advance in drug delivery is the ability to deliver and transfect nucleic acids, including mRNA, siRNA, and DNA. The ability to directly encode cells to produce specific proteins or silence genes is powerful because it enables the direct programming of cells, including the use of CRISPR (clustered regularly interspaced short palindromic repeats) components. Although viral vectors can be very effective in transfecting genetic material, they also pose health and safety risks, as well as issues of scale for manufacturing, reproducibility, and tunability. Challenges to the delivery of nucleic acids are multifold; they include the need to compact a very large and highly negatively charged macromolecular species to a size compatible with cell uptake and the need to create a protective coating upon encapsulation of the nucleic acid to protect against exposure to proteases in the bloodstream. It is also important for systemic delivery approaches that the encapsulating materials system have a neutral or negative charge because of the cytotoxicity and protein opsonization resulting from positively charged nanomaterials. Ultimately, key susceptibilities for delivery of these biologic systems have been degradation of the RNA/DNA molecules themselves due to the presence of RNAse or DNAse enzymes in the body. Recent advances in func-
tionalization of the nucleic acids can render siRNA cargoes fairly inert to breakdown under physiological conditions; however, although mRNA can be protected with functional groups, it remains more susceptible to breakdown and requires packaging that protects against degradation. Additional issues requiring attention are the targeting of nanocarriers to desired organ or cell types and the ability to direct trafficking of the nanoparticle to the cytosol or nucleus, where the cargo can be available for direct transfection or translation.
Cationic lipid nanoparticles (LNPs) have been found to exhibit the above properties while generating stable complexes that protect nontarget cells and tissues from exposure to the nucleic acid cargo during distribution throughout the body. The positive charge of the lipids interacts with negatively charged RNA backbones to generate uniform nanoparticles, usually with a net positive charge. If the cationic lipids are combined with pegylated lipid molecules, the PEG can act to dilute and shield positive charge, making the resulting structures increasingly stable while avoiding interactions with serum proteins and circulating cells, such as monocytes, that lead to clearance and destabilization. Key to the successful delivery process is the need for the cargo to exit the endosome as it buffers to lower pH. Cationic LNPs are the basis for effective endosomal escape, enabling release of RNA or DNA to the cytosol; it is thought that the lipids aid in compromising the endosomal membrane and lead to its disruption and release. The first LNP therapeutics were introduced by Alnylam as an siRNA therapeutic and involved a cationic lipid complexed with a chemically stabilized RNA interference (RNAi) molecule. Significant advances in the composition and structure of the lipids used for LNPs enabled the development of the Moderna and Pfizer mRNA vaccines for COVID-19 and form the basis of a powerful platform for RNA medicine. Novel and improved formulations and production of LNPs will be important for future vaccine manufacturing.
There are some limitations to the use of cationic LNPs. Lipids tend to traffic to specific regions of the body, including the liver, because of the apolipoprotein E (ApoE) receptor present on liver cells, and it can be challenging to design lipids with appropriate biodistributions for targeting other organs following intravenous injection. Lipids also provide fewer chemical handles for introducing smart or responsive chemistries, and they do not allow for staged or more complex release systems. Polymeric systems excel in providing a large design space while enabling the presence of high-charge-density segments by using block or segmented block copolymers or polyelectrolytes. Although some polymers have been found to be highly effective transfection agents, a trade-off between efficacy and toxicity has resulted from the impact of their high positive charge. One of the important biomaterials challenges in the upcoming decades will be the discovery and design of synthetic vectors that can rival the transfection efficiencies of viral delivery while remaining highly safe.
ELECTRONIC MATERIALS
Chemical engineers have played a central role in the discovery, design, and production of the materials (e.g., polymers, semiconductors, glasses) needed to create electronic devices. The growth in demand for these devices, and thus these materials, shows no signs of slowing. The development of new materials for these applications will require
sophisticated research and development, as discussed here, and will likely require elements of AI and data analytics for the materials development (see Chapter 8).
Semiconductor Manufacturing
The common process steps in manufacturing semiconductor devices are insulator deposition, lithography, etching, wet cleaning, metallization, and chemical–mechanical planarization, although not necessarily in that order (Table 7-1). Common challenges for these steps include ever-increasing purity requirements in response to increased upstream/downstream process sensitivities, environmental considerations in response to global requirements and compatibility, means of maintaining consistent manufacturing and packaging processes, and approaches for managing and improving global distribution. There can also be challenges specific to individual molecule or formulation types, such as the stability of the formulation and its sensitivity to air and the safe handling of flammable or toxic materials.
The critical processes for materials manufacturing include synthesis, purification (including distillation and filtration), formulation blending, and packaging and materials compatibility. Each of these processes may be considered during the discovery phase of a new material but are fully accounted for during the transition to mass production.
Several trends are expected to continue. First, the number of new materials being introduced to the semiconductor and adjacent electronic industries will continue to increase, and more rapid molecule or formulation identification will be required to meet the industry cadence. In addition, some historically important processes may become obsolete because of environmental restrictions and a lack of supply due to supply chain interruptions (especially in the case of rare earth elements) or general shortages (as in the case of helium).
Second, materials will need to be increasingly pure, with the purity levels of metals reaching the low parts-per-trillion range, and greater synthetic process control will be needed to reduce already low-level organic and inorganic impurities. The enhanced purity requirements will drive improvements to in-line analytical process monitoring and real-time process control, as well as other advances in manufacturing. Chemical engineers can play a role in each of these areas. Finally, the markets for most major materials used for semiconductor manufacturing are expected to grow (Tremblay, 2018), driven by increasing use of materials in manufacturing of both logic and memory devices, as well as overall industry growth.
Challenges in Electronic Materials Discovery
Research and development of electronic materials occur in sequence but have different inputs, outputs, timelines, and risks. For suppliers of materials to the electronics industry, the input to the research process is an established material need from device makers, and the output is enabling knowledge, ultimately in the form of a product concept to satisfy that need. The product concept is the input for the development process, which determines how this concept can be realized as a salable product manufactured economically and reliably at scale.
TABLE 7-1 Primary Semiconductor Manufacturing Processes, Common Materials Used in Each Process, and Chemical Engineering Processes Required
Manufacturing Process Step | Type of Processing | General Material Classes | Current Material Challenges | Related Chemical Engineering Processes |
---|---|---|---|---|
Deposition |
|
|
|
|
Etching and dopant gases |
|
|
|
|
Lithography |
|
|
|
|
Wet cleaning |
|
|
|
|
Chemical mechanical planarization |
|
|
|
|
SOURCE: Internal knowledge from EMD Electronics, 2021.
The unmet current and future needs for advanced materials for the electronics industry are being addressed through close collaboration with foundries and independent device manufacturers (IDMs), precompetitive consortia, and industry roadmaps. Industry roadmaps, now in their third generation (Gargini et al., 2020), take the longest view in identifying key trends, industry challenges, potential solutions, and required innovations over the next 15 years. These roadmaps have been invaluable in accelerating industry advances by advancing the best technologies to keep pace with Moore’s law. Material suppliers with appropriate resources can confidently make long-term strategic research and business decisions based on these roadmaps.
Since the formation of SEMATECH as an industry–government partnership to maintain U.S. leadership in the semiconductor industry (Irwin and Klenow, 1996) and its evolution into an international precompetitive consortium (Carayannis and Alexander, 2004), precompetitive strategic partnerships have been instrumental in the electronics industry to advance the technologies needed to realize industry roadmaps (Logar et al., 2014). Medium-term needs for materials suppliers can be understood through participation in these consortia. Near-term manufacturing needs can be understood only through close collaboration with the foundries and IDMs. Collaborative innovation within the semiconductor industry confers an advantage despite the challenges of maintaining relationships and controlling proprietary information (Kapoor, 2012). Materials suppliers can receive timely feedback on new product performance and integration issues, which enables faster implementation of new processes for device manufacturers (O’Neill and Zheng, 2019).
Once a material need has been specified, the research process of discovery begins. That process has evolved over the past several decades but follows the basic cyclical scientific method of relevant field study, hypothesis development, testing, and assessment. In the past, this cyclical method was a slow, trial-and-error process. Leveraging the arrival of more powerful computers and improved theoretical calculations in the second half of the last century, the current so-called third age of quantum chemistry now enables calculations at least as accurate as the results of physical experimentation (Langhoff, 1995; Richards, 1979). Thus, there is now a rational approach to the design of materials, one that entails creating new molecules with desired properties using predictions from models based on atomic or molecular structures. The accuracy and utility of these models are directly tied to the rapid scaling of computational power, which itself was enabled by the discovery of new processes and materials used by the semiconductor industry. These improvements led to better and faster computational results, such that screening experiments can be conducted reliably in silico (Hafner et al., 2006; Hautier et al., 2012), limiting the synthesis targets for physical experimentation to compounds or mixtures with the best prospects of achieving the material needs of the industry. Additionally, laboratory experimentation has been enhanced by the commonplace computer control of experimental apparatus and analytical instrumentation (Ford, 1982). Thus, advanced computing has increased efficiencies in the testing phase with high-throughput screening and combinatorial material synthesis. These approaches revolutionized pharmaceutical research but have now been demonstrated for discovery of new materials in semiconductors, especially
when the new material can be synthesized and evaluated as a thin film (Takeuchi et al., 2005).
Strategies for Development of Electronic Materials
Chemical engineering merges chemistry fundamentals and material physics with the hardware and equipment required to produce the desired commercial chemical products. The electronic materials manufacturing industry elevates this challenge to a new level not experienced with the manufacture of many traditional chemical products. Comparison with the evolution of the pharmaceutical chemical industry offers a useful guide for the advancement of the electronic materials industry.
Purity and product uniformity have improved throughout the progress of the electronic materials industry. Early in the history of integrated circuit (IC) manufacturing, materials were used as they were available in the standard reagent and commodity chemicals market. Later, IC manufacturers, using statistical tools to characterize chip-manufacturing performance, drove the quality and specifications for materials far beyond what was generally available. Thus, the development of purification technologies is a primary driver for new products across the industry, and also drives the need for continuous quality verification using process analytical technology and supporting statistical tools that must be upgraded to handle the massive amounts of data generated with automation. The future will look more and more toward AI to enhance problem solving and material and process design (see Chapter 8).
Materials used in the electronics industry are constantly changing in response to the new requirements of manufacturers. Many of these materials are unique in structure, can be very hazardous, and require customized reactors and processes to produce. These reactor and separation unit operations require a great deal of creative technology design. The development of robust processes can be accelerated when capital costs are kept low and process equipment can be kept small. Process-intensification methods, modular continuous reactors, microreactors, and purification platforms can speed up product commercialization, with assets prepositioned globally (Tian et al., 2018).
Many of the raw materials used to manufacture electronic chemical products are available only as commodities with limited purity, far below the necessary requirements. Efforts are increasingly focused on the development of purification technologies using traditional and novel purification equipment, methods, and schemes. Additionally, the stringent requirements for product purity require that suppliers of electronic materials evaluate and characterize the subtle and increasingly important impact of equipment materials of construction on process chemistry. The industry organization SEMI provides standards for purity, analytical methods, and processing for many raw materials.1
Metallic impurities are notoriously problematic in all electronic materials, limiting the materials of construction suitable for manufacturing and separation equipment. Even extremely low levels of surface corrosion can lead to unusable products. Polymeric materials, on the other hand, can leach low levels of unbound polymers or plasticizers.
___________________
Glass reactors and process equipment can be vulnerable to extremes in pH, which can cause leaching of silica, boron, and metals. Surface science is a critical and ever more important discipline, and chemical engineers will play a role in the development of new products and processes for this industry.
Ion-exchange resin processing is commonly used to remove metal impurities in aqueous and organic raw materials. The diverse chemical functional groups available on resins provide a wide range of metal-removal selectivity. The choice of resins and combinations of resins can be made specific to the metals (and their oxidation state) to be removed. Preparation and pretreatment of the resin bed are important to reduce polymer contamination. Materials often need to be diluted before processing to achieve the necessary removal purity and then reconcentrated (Alexandratos, 2009; Silva et al., 2018).
Other purification methods include many variations on distillation, filtration, and extraction. They are usually applied for small-scale continuous processes, and include packed-column distillation, molecular distillation, wiped-film and spinning-band distillation, sublimation, liquid extraction using Karr columns and rotating-disc columns, crystallization, adsorption for both liquid- and gas-phase materials, ion exchange, crossflow and flow-through filtration on novel modified membranes and plastics, and simulated moving-bed chromatography. Hydrogen peroxide, for example, is a critical material used in chemical mechanical polishing, and a new approach to reverse osmosis is proposed as a novel method for purification (Abejón et al., 2010; Gao et al., 2017).
Cleaning procedures as they pertain to such equipment as piping, pumping, and storage vessels are frequently underappreciated. Especially in the pharmaceutical, fine chemical, and electronic chemicals industry, cleaning of equipment between changes in product, raw material lots, or batches is often a complex process. Cleaning processes require detailed metrics, and in many cases may be as complex and time-consuming as the chemical synthesis or purification process. Chemical surface preparation of unit operation equipment and packaging containers will become a project in surface science and a subset of the overall process development.
CHALLENGES AND OPPORTUNITIES
Chemical engineers can contribute to the materials development domain across a range of material types and applications. In particular, they have a unique role to play in the continued development of polymer science and engineering because of their understanding of chemical synthesis and catalysis, thermodynamics, transport and rheology, and process and systems design. Chemical engineering is the logical home for research and development of complex fluids and soft matter. The combination of molecular-level understanding and thermodynamic and transport concepts yields important insights and enables advances. The science and application of nanoparticles by chemical engineers in both industry and biomedicine are rapidly accelerating, offering the opportunity for application breakthroughs. Chemical engineers play an essential role in advancing the development of biomaterials for both regenerative engineering and organ-on-a-chip technology, and chemical engineering principles are at the heart of understanding and improving targeted drug delivery both spatially and temporally. As the United States has
lost dominance in the area of semiconductor processing, chemical engineering expertise around reactor design, separations, and process intensification has become critical to the success and growth of the electronic materials industry.
Recommendation 7-1: Federal and industry research investments in materials should be directed to
- polymer science and engineering, with a focus on life-cycle considerations, multiscale simulation, artificial intelligence, and structure/property/processing approaches;
- basic research to build new knowledge in complex fluids and soft matter;
- nanoparticle synthesis and assembly, with the goal of creating new materials by self- or directed assembly, as well as improvements in the safety and efficacy of nanoparticle therapies; and
- discovery and design of new reaction schemes and purification processes, with a steady focus on process intensification, especially for applications in electronic materials.