7
Health, Medicine, and Technology
The project of writing this report has overlapped almost completely with the worldwide COVID-19 pandemic. Millions of people around the world have died, and the isolation required to restrict the spread of the disease has taken a severe toll on society. A bright spot in this dark chapter of human history is the extraordinary response of the scientific community. As always with the solution of major technological problems, what success the world has had in combating COVID-19 has been woven from many threads, but many results, ideas, and methods that have emerged from the biological physics community proved foundational for this success. The society has effective responses to the pandemic because many scientists focused their extraordinary skills on the problem, but also because they could build on an extensive intellectual infrastructure. This infrastructure supports much more than our response to the pandemic, reaching into almost every aspect of health and medicine, and many areas of technology more broadly. This chapter explores some of this infrastructure, how parts of it emerged from the biological physics community, and how it has influenced the progress of human health, medicine, and technology more generally; an overview is given in Table 7.1.
The practice of medicine has been revolutionized by our ability to see what is happening inside the body and in isolated cells, on scales from single molecules to whole organs. Our understanding of disease, and our ability to respond to it, have been profoundly affected by our ability to resolve the structures of the relevant molecules down to the positions of individual atoms, and to design new molecules that emulate, extend, or block the functions of those that occur naturally. Our understanding of the basic mechanisms of information flow and control in living cells
TABLE 7.1 Broad Societal Impact Through “Physics of Life” Intellectual Infrastructure
“Physics of Life” Topics: Intellectual Infrastructure Supporting Health, Medicine, and Technology | Capabilities or Promise of Biological Physics | Connected Topic, Field, or Application |
---|---|---|
Imaging, diagnostics, and treatment | See what is happening inside the body and in isolated cells, on scales from single molecules to whole organs. | Medical physics; medical diagnoses. |
Molecular design | View structures of the relevant molecules down to the positions of individual atoms, and design new molecules that emulate, extend, or block the functions of those that occur naturally. | Drug design. |
Synthetic biology | Engineering cells that can be harnessed to address problems. | Personalized medicine; synthesis of clean fuels. |
Predicting and controlling evolution | Creating a vaccine by predicting the future trajectory of the virus’s evolutionary change. | COVID-19; seasonal flu. |
Biomechanics and robotics | Enables building truly new things, and predicting how these things will behave; engineering and design guided by the physical principles at work in living systems. | Robots. |
Neural networks and artificial intelligence | Emulate human and animal performance at challenging tasks, ranging from walking on rough terrain to understanding language. | Ongoing revolution in artificial intelligence. |
created the opportunity for a new kind of engineering, using these mechanisms as building blocks in much the same way that computer chips use transistors, capacitors, and resistors; these engineered cells can be harnessed to address problems as diverse as personalized medicine and the synthesis of clean fuels. While popular accounts of evolution often emphasize that it is driven by random mutations, creating a vaccine against next year’s seasonal flu requires predicting the future trajectory of the virus’ evolutionary change, and there has been remarkable progress on this fundamental problem.
Many of these different themes, and more, came together as the scientific community responded to COVID-19. As soon as SARS-CoV-2 was isolated there were rapid efforts to determine the structures of crucial molecular components, including the infamous spike protein that enables viral entry into cells. Methods for single molecule counting and visualization provided extraordinarily sensitive probes for the presence of the virus, and sequencing methods focused attention on parts of the antibody repertoire that were central to an effective immune response. Methods for tracking and predicting the evolution of influenza virus, which had come
directly out of the biological physics community’s engagement with evolutionary dynamics, were immediately adapted to the new virus and became the worldwide standard. In particular, these methods led to the first detection of community spread of COVID-19 in the Seattle area as early as January 2020, months before the scale of the public health crisis facing the United States (and the world) was evident. A generation of work that connects the biological physics community with ecology and epidemiology resulted in the rapid development of effective models for spread of the virus, within which the impact of different public health measures could be assessed. This provided direct input to public health policy in the United States and around the world, including the difficult decisions to shut down institutions and whole states. Acting locally, many members of the biological physics community have been central to their institutions’ efforts to expand testing capabilities, helping to provide infrastructure to support a safe return to in-person teaching.
The biological physics community studied the spread of droplets experimentally, using particle image velocimetry and other physics techniques, and theoretically, using fluid dynamics simulations. Such studies provided evidence that aerosols are extremely important in transmission of the virus, at a moment when this was controversial. This technical work was adapted into accessible videos to demonstrate the efficacy of masks in blocking droplets to a larger audience. Finally, the ingenuity of biological physicists has been directed toward developing substitutes for medical supplies in short supply during peaks of the pandemic, such as N95-like filter materials made using repurposed cotton candy machines—inexpensive workarounds that may be useful in future pandemics. The community also developed and circulated designs for cheap and easy-to-build ventilators and oxygen concentrators that can be constructed from commonly available parts.
The pandemic inspired many members of the biological physics community, as with the scientific community more broadly, to engage with a problem of immediate concern to the society at large. The evidence of success, and the intellectual excitement that surrounds such an urgent problem, will have lasting impact. In addition, biological physicists have directed efforts toward many other public health problems beyond the COVID-19 pandemic. One example is the recent development of microscopes made largely out of folded paper that cost under a dollar and have a resolution of 2 microns. Similarly, centrifuges can be made from paper, string, and plastic for 20 cents that can spin medical fluid samples at up to 125,000 rotations per minute. Although currently used primarily as tools for teaching, one can imagine that these inexpensive instruments will have broader impact in the developing world.
One profound consequence of understanding physics is that it enables building truly new things, and predicting how these things will behave. While the lightbulb could be invented by trial and error, building a working smartphone requires design, and depends on our understanding of how electrical currents flow in the
millions of devices that are packed into each computer chip. Progress in biological physics holds the promise of engineering and design guided by the physical principles at work in living systems, beyond the emulation or harnessing of particular mechanisms. Examples include robots and computers that emulate human and animal performance at challenging tasks, ranging from walking on rough terrain to understanding language. Although there has been revolutionary progress, it is reasonable to expect that addressing the more basic scientific challenges outlined in Part I of this report will lead to even more powerful and life-changing discoveries and inventions in the future.
IMAGING, DIAGNOSTICS, AND TREATMENT
Since the first microscopes were built in the 1600s, our ability to visualize or image the phenomena of life has been central to scientific progress. As is clear from many examples in Part I of this report, progress in imaging also is central to today’s biological physics. Importantly, these same imaging methods also have revolutionized the practice of medicine. These developments have spawned major industries and shaped societal expectations regarding medical care. The impact of biological physics on human health can be discerned even before a baby is born, when a fetal heartbeat is detected by Doppler sonography and the fetus is imaged by ultrasound tomography. If the parents’ fertility has been examined, then sperm motility will likely have been assessed with technologies such as microfluidic methods. Later, when a child injures a limb or requires a dental cavity to be filled, X-ray imaging will be used to inspect for broken bones or identify tooth decay. As individuals age, routine clinical screening may involve optical endoscopy, flow cytometry, and electrocardiography—all physical methods. Optical coherence tomography and the fundus camera provide assessments of retinal health. In patients with treatment-resistant depression or chronic pain, transcranial magnetic stimulation can often provide relief. In those with suspected neurological disorders and diseases, physical methods such as magnetic resonance imaging (MRI), positron emission tomography (PET), electroencephalography (EEG), or electromyography (EMG) may be critical for diagnoses. When cancer is suspected, flow cytometry and X-ray computed tomography or PET imaging may be used for diagnosis, while light microscopy and fluorescent histopathological stains may be used for detailed characterizations of a cancer and its level of invasiveness. Cancer treatment may involve the targeted delivery of X rays, gamma rays, or even protons to solid tumors. Microfluidic and gene sequencing assays may be used to detect circulating tumor cells. Emerging, personalized cancer therapies involve gene sequencing and genome editing of immune cells, guided by increasingly sophisticated theoretical approaches.
Medical physics largely emerged after the discovery of X rays and their preferential absorption by the body’s hard tissues, chiefly in the musculoskeletal system,
in contrast to the far greater X-ray transparency of soft tissues. Within only a few years after this discovery, medical researchers began investigating the use of X-radiography for non-invasive imaging of the body. Further development and refinement of X-ray–based medical imaging continues to the present day. The invention of tomographic three-dimensional X-ray imaging, now widely known as X-ray computed tomography (CT), is used in hospitals worldwide and has an essential role in a wide range of image-based diagnostics; an example is in Figure 7.1. Ongoing research in this area remains vibrant and includes pursuits such as the innovation of new solid-state X-ray detectors, image processing techniques to empower new medical diagnostics, and machine-learning based methods for automated detection of tissue abnormalities.1
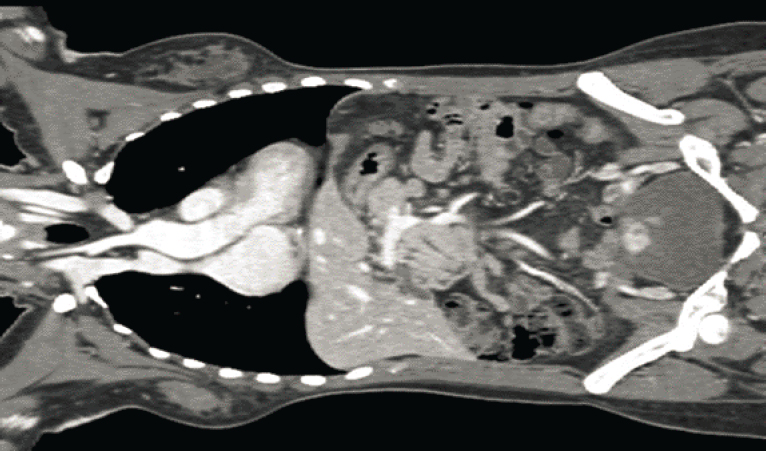
___________________
1 Anecdotally, if you are reading this report soon after its release, you probably have experienced some of this revolution. As a child, your X rays were likely taken with a large instrument that projected an image onto photographic film. The film was developed and stored at your doctor’s office for future reference. Today, if you need a chest X ray, it is likely that you stand with a compact X-ray source in front of you and an “area detector” behind you. The detector, adapted from technologies used in physics laboratories, registers an image electronically and transmits it directly to a computer. No more film, no more file cabinets. This reflects progress in computers and information technology more broadly but would be impossible without the new X-ray sources and detectors.
Other portions of the electromagnetic spectrum also have important roles in medical imaging. For instance, gamma ray cameras are scintillation cameras with key roles in nuclear medicine (see Figure 7.2). By injecting a patient with a radiopharmaceutical—a pharmacologically active compound that will bind to a specific molecular target and emit gamma rays—radiologists can use the gamma ray camera to determine where in the body the radiopharmaceutical has accumulated. This approach has played important roles in cancer diagnosis and assessment, as well as in the diagnosis of abnormalities of the thyroid, heart, and lungs. Notably, the speed of imaging can be sufficiently fast to allow tracking of a radiotracer through the beating heart, enabling inspection of the contractile pattern. As with X rays, gamma-ray imaging can also be done in a tomographic manner, in this case called single photon emission computed tomography (SPECT).
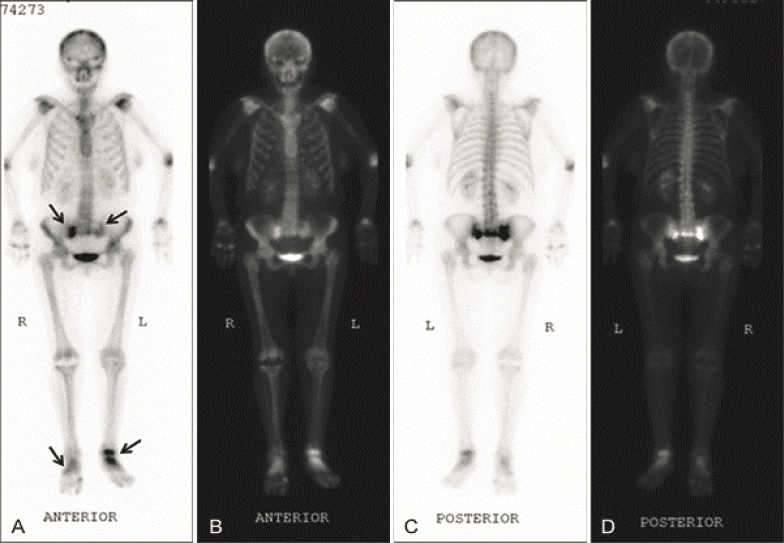
Gamma rays also play a critical role in PET, another widespread form of noninvasive medical imaging. The patient is administered a beta-emitting radiotracer, which localizes to tissues or cells with selective characteristics. Typically, this compound has a specific biological activity or receptor, allowing physicians to target a particular biological process (such as in metabolism) or dynamic within the body. This stands in contrast to X-ray imaging, which is usually better suited for revealing tissue morphology, although exogenous X-ray contrast agents can be used to track physiological processes. Due to their typically brief half-lives, PET radiotracers are often produced at cyclotrons at or near medical facilities where PET imaging is done. Upon emission within the body, positrons generally travel only a short distance within tissue before annihilating with an electron to produce a pair of gamma ray photons traveling in opposite directions. The coincident detection of this photon pair enables a determination of a line along which the emitter must have resided, and by rotation of the detector array, a three-dimensional tomographic image can be computed.
Today, PET imaging is a workhorse technique for cancer diagnosis. Moreover, thanks to its ability to target specific biological processes of interest—often limited only by the ability of radiochemists to produce a suitable tracer—PET also has a forefront role in the growing radiological subfield of molecular imaging. For instance, neuropsychiatry has used PET to examine differences in the distributions of dopamine receptor subtypes in the brains of normal versus depressed human subjects. Related methods for PET imaging of the dopamine system allow the diagnosis of Parkinson’s disease. Ongoing research seeks new radiotracers, new detectors, and new methods of image analysis.
Like X-ray CT and PET, MRI is also a computed tomography. However, it does not require the use of ionizing radiation and can be used to inspect soft tissue details with an exogenous contrast agent, leading to unique diagnostic roles in neurology, cardiology, orthopedics and sports medicine, and hepatology. MRI is based on the principles of nuclear magnetic resonance: Individual atomic nuclei, when placed in a strong magnetic field, can absorb and emit radio waves. The frequency of the waves depends on the field, and this means that by applying a magnetic field that varies in space one can map the positions of the atoms, today with a resolution of roughly 1.5 mm. The simplest choice, and the one most widely used in medical applications, is to probe the hydrogen nuclei in the water that comprises a large part of all living material; other nuclei can also be chosen, such as for NMR spectroscopy studies of metabolism, but these are far less common in a diagnostic context. By choosing different sequences of radio wave pulses, MRI signals become sensitive to different aspects of energy exchange or relaxation (T1 and T2) between individual hydrogen atoms and their surroundings, highlighting specific tissue features. MRI contrast agents, which typically involve chelates of gadolinium, have further expanded the range of medical applications beyond those
in which the tissue of interest naturally exhibits suitable MRI contrast. Magnetic resonance images can also be sensitive to the functional activity of tissues, leading to opportunities in visualizing the human brain in action, as noted in Chapters 3 and 6, as well as to measuring concentrations of specific molecules down to millimolar concentrations and millimeter resolution.
Acoustic waves underlie ultrasound imaging, a widely used means of imaging the body’s soft tissues in a non-invasive manner. A modern ultrasound probe typically comprises both acoustic emitters and an array of detectors. An acoustic impedance matching gel is applied between the probe and the tissue to minimize unwanted loss of acoustic energy at the tissue surface. Ultrasound waves enter the body, reflect off body tissues, and can be detected upon exiting the body. The round-trip “time of flight” for this process enables a determination of the depth at which reflection occurred in the tissue. Image contrast comes from the variability in the acoustic reflectivity of different tissue types; phased arrays enable tomographic imaging. The imaging resolution is limited by the acoustic wavelength and typically is sub-millimeter; unfortunately, higher-frequency ultrasound waves enabling finer resolution attenuate more readily in biological tissue and cannot penetrate as deeply. New forms of ultrasound imaging continue to emerge, enabled by progress in the design of emitter-detector arrays, new forms of detectors, and new computational engines for real-time image determination. For example, an emerging form of ultrasound imaging is photoacoustic imaging, which relies on the faint ultrasound emission that occurs during non-radiative decay of an optical excitation. Although the signals involved are weak, photoacoustic imaging has generated excitement because it can combine the molecular specificity of an optical probe with the imaging depth of ultrasound. Of course, optical emissions also have an enormous role in medical imaging. Nearly all of modern pathology rests on the ability to examine excised tissue specimens under a light microscope. Classical histopathological stains, such as hematoxylin and eosin (H&E), bind to specific tissues, alter their optical absorption spectra, and thereby enable a majority of pathological diagnostics that remain in common use. More advanced pathological techniques involve the use of fluorescence stains, such as immunofluorescent labels or fluorescent in situ hybridization (FISH) probes. These are the methods that have been pushed to the point of counting single molecules and surveying the expression of many genes, as in Figure 2.6. Unlike H&E, these fluorescence-based methods in pathology have the advantage of revealing specific proteins or RNA sequences in the tissue specimen, which can enable more precise diagnoses. While not strictly an imaging technique, fluorescence-activated cell-sorting (FACS) combines immunofluorescence and flow cytometry and has also enabled major advances in medical diagnostics, particularly in hematology, immunology, and cancer biology.
Beyond the benchtop microscope, visible light underlies nearly all of endoscopy, which is widely used to image the gastrointestinal system, lungs, bladder,
throat, ears, brain, and other body tissues. The invention of fiber optics was a crucial development in physics that made such diverse diagnostics possible. Today, endoscopes are an essential component of not just diagnosis but also minimally invasive surgery, as they can be equipped with a working channel that allows in situ specimen acquisition and the introduction of surgical tools through the endoscope itself. Equally important is the surgical microscope, which comes in many forms and is used in diverse surgical procedures. Today, leading-edge research aims to create new endoscopes capable of advanced forms of optical imaging, for example to achieve cellular resolution or to use nonlinear optical mechanisms, as well as surgical microscopes with robotic manipulation capabilities.
Ophthalmology owes a tremendous amount to the field of optics, without which it would be impossible to provide proper eyeglasses or to perform vision correction surgeries by using an excimer laser to modify the shape of the cornea (LASIK) so as to ameliorate the eye’s optical aberrations.
Ideas from biological physics have also been pivotal to a wide range of prosthetic devices that can be used to facilitate body function or ameliorate dysfunction. For those who have suffered the loss of a limb, biomechanics has led to prosthetic arms and legs with increasingly impressive mechanical properties. Cardiac pacemakers facilitate proper timing of the heartbeat by providing electrical synchronization when the heart’s electrical conduction system is impaired. For Parkinson’s disease, an electrical neurostimulator may be implanted into the brain to reduce tremor. Hearing loss may be counteracted with a digital hearing aid; if deafness is severe, a cortical prosthetic may be implanted into the inner ear, allowing direct electrical stimulation of auditory afferents in response to sound. Emerging methods for retinal prostheses promise to restore sight to the blind. There are even emerging brain-computer interfaces, which allow direct communication between the human brain and external electronics for people who are fully paralyzed. These developments depend both on advances in technology and on theoretical ideas about the representation of information in the brain.
Some sense for the liveliness of this enterprise comes from the continued emergence of startup companies based on technologies that have emerged from the biological physics community, as described in Box 7.1.
MOLECULAR DESIGN
Biological molecules perform an astonishing array of functions. It is an old dream to harness and adapt these functions for engineering purposes. One approach is to use an evolutionary strategy, generating large numbers of variants, selecting for molecules closer to the desired function, and repeating. This approach has been made practical, and was recognized by a Nobel Prize in 2018. A different approach is to actually design molecules, in the same way that humans design other engineered objects.
A natural target for molecular design are protein molecules. Protein functions are determined to a large extent by their three-dimensional folded structures, and structures are determined by amino acid sequences. So the targets of design are particular structures, while the space of possibilities is the set of amino acid sequences. Protein design requires us to understand the sequence/structure mapping, a problem that has appeared in previous sections of this report.
Proteins are unusual because many of them are polymers that fold into well-defined compact structures. A fundamental problem in modern biological physics is to understand how this is possible (Chapter 3): What is it about the amino acid sequences of real proteins that allows for more or less unique structures, avoiding the competing interactions that lead to glassy behavior in typical random sequences? There is also a practical version of the problem (Chapter 6): Given a sequence that is known to fold, what is the resulting folded structure? Protein design is yet a third version of the problem, inverse to the practical folding problem.
The exploration of principles underlying the emergence of folded proteins provides several important hints about design. First, in a successful design, the energy landscape for the protein will have a form that assists rather than frustrates the search for the folded structure, funneling the molecule toward its final
configuration as in Figure 3.4. Second, real structures are highly designable, so that many sequences map to nearly the same structure. Third, within this large set of sequences, there is a kind of smoothness so that it is possible to capture the structural constraints by keeping track of only pairwise correlations among amino acid substitutions.
The success of pairwise models means that one can generate new sequences that will fold into known structures, as discussed in Chapter 3. It is important that these new sequences can be very far from the original sequences; as such, this approach allows us to probe the sequence space globally rather than just locally. The funneled structure of the energy landscape suggests that, although high dimensional, the folding of a protein is simpler than it might have been without the competing local minima that characterize computationally hard optimization problems in computer science and physical systems such as glasses that fail to find their minimum energy configuration in a reasonable time. In a startling development, the problem of predicting protein structure from the amino acid sequence has inspired the development of a direct machine learning approach, “AlphaFold,” which achieves a precision close to that of experimental structure determination by X-ray crystallography.
A corollary of the ideas mentioned above is that the number of protein sequences seen in nature today is much larger than the number of protein folds, or folding motifs, that have been found by studying protein structures. It is not clear just how strong this limitation really is. Has evolution discovered all the possible folding motifs, or can we synthesize new ones? Successful efforts aimed at discovering new motifs have been very limited. One of the first successes toward this goal has been the protein called Top7 (see Figure 7.3), which is able to fold into a unique structure but does not have many of the properties of real proteins. Although much effort has been dedicated to de novo protein engineering, genuinely new folds remain rare, and searches for new functionality have focused on combining known motifs. Basic questions about the universe of possible protein structures are closely tied to our hopes for further progress in the practical problem of protein design.
Beyond protein design, structure-based drug design (SBDD) is an integral element of most pharmaceutical industries developing therapeutics or vaccines. SBDD refers to the design and optimization of chemical or protein entities that interact with the molecular machines in the cell in order to select those most suitable for further optimization as potential clinical therapeutics (i.e., drug candidates). The basic approach is to develop a detailed understanding of the three-dimensional structure of the protein target, its interaction with the potential drug, and how the shapes and charge of the protein and drug affect their interactions. These fundamental atomic relationships correlate to the efficacy and safety of the drug in clinical trials.
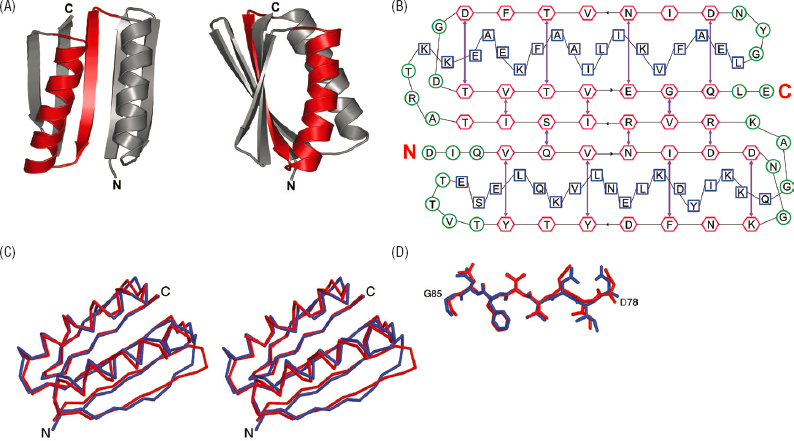
The basic science underlying this process is supported by proteomics, structural genomics, information technology, cloning, expression, and purification of protein targets, as well as by high-throughput crystallography, NMR, and most recently cryo electron microscopy (cryoEM). The revolution in computer technology has also been integral to all of these efforts while also ushering in the prospect of in silico identification and optimization of drug targets, as with protein design above.
The year 2020 has especially highlighted the value of SBDD. The COVID-19 pandemic put a brief pause on the activities of most labs focused on protein structure and SBDD as the pandemic shut down normal activities, but this was very rapidly followed by a massive retooling to devote the full force of these efforts toward understanding the structure and function of SARS-CoV-2. Research groups focused on determining structures using X-ray crystallography, NMR, cryoEM, or computational methods turned their full attention to the machinery of the novel
coronavirus; see, for example, Figure I.7. Efforts have been focused on understanding the “trimeric spike,” the invasion mechanism of the virus and a target for vaccines and antibody therapies, as well as the basic viral replication mechanism, a target for small molecule drug therapeutics. In addition, it was fundamental work to understand the structure of the earlier coronavirus spikes that allowed for the possibility of rapid design of vaccines within days of the viral sequence being published. Current efforts are contributing to the development of second-generation vaccine candidates and potentially also a universal coronavirus vaccine that will safeguard us from future pandemics.
Can one go beyond SBDD and utilize the protein’s full energy landscape when designing new drugs? SBDD is driven by the idea that binders with higher binding affinity to a target should be more efficacious than those with lower binding affinity to the same target. This approach is incomplete and therefore the ability to understand the kinetics of drug binding together with protein motions is now becoming a new challenge. To elucidate these relationships, energy landscape theory has been generalized to create a computational framework that is able to construct a complete ligand-target binding free energy landscape. In another example, this strategy has been generalized to develop therapeutic drugs that regulate multiprotein complexes. The challenge here is to identify molecular compounds that can impact the protein-protein binding interface. Success requires identifying these binding interfaces and designing compounds that compete with these interface interactions. The open question is when these expanding ideas from physics will allow for the design of drugs that could not be discovered by the current strategies.
SYNTHETIC BIOLOGY
Synthetic biology emerged in the early 2000s as a number of researchers, many with physics and engineering backgrounds, took an interest in designing synthetic living systems that either mimicked real ones or behaved in completely new ways. The goals of this type of research are both practical, leading to useful technologies, and fundamental, to better understand what is life by attempting to build it. Research in synthetic biology is powered by technological advances in genetic engineering, live cell imaging, sequencing, protein engineering, and so on, and often pursued in interdisciplinary teams that bring together physicists, engineers, biochemists, and cell biologists.
As with almost every other scientific discovery, the discovery of gene regulation provided the means and impetus to assert human control over nature, in this case the genetic networks in cells. Starting with early proof-of-principle experiments that demonstrated how simple toggle switches and oscillators could be fashioned from repurposed bacterial genes and their control elements, today’s synthetic genetic networks are beginning to approach the complexity of electronic ones.
These approaches could be applied to develop designer cells that seek and destroy pathogens in the human body, or to produce useful chemicals for medicinal or biofuel applications.
Uploading DNA-based circuits into cells so as to repair a diseased state, as shown in Figure 7.4, is one approach to personalized medicine, where a treatment is rationally designed based on the individual characteristics of a patient. In attempts to combat the rise of drug-resistant bacteria, viruses are being designed that can kill the bacterial pathogens. Bacteria, on the other hand, have been engineered to do our bidding and target and invade cancer cells. While these initial successes have only occurred in carefully staged laboratory conditions, the hope is that clinical applications are soon to follow.
Genetic manipulation of algae is being used to develop a new generation of biofuels. Algae are single celled organisms that live in an aquatic environment and can convert carbon from sugars or carbon dioxide into biomass. Synthetic biology approaches are being used to make algae that produce more biofuel, or that make the extraction process easier, for example by introducing mutations that decrease cell wall thickness and thereby make lipids and other biomolecules more accessible.
Antecedents to the synthetic biology revolution are biofuels produced from starch, sugar, animal fats, and vegetable oils, which are playing an increasingly large role in our energy landscape. Ethanol, a first-generation biofuel made primarily from corn, is one of the main biobased products produced worldwide and is present in more than 98 percent of the gasoline sold in the United States. Starch content,
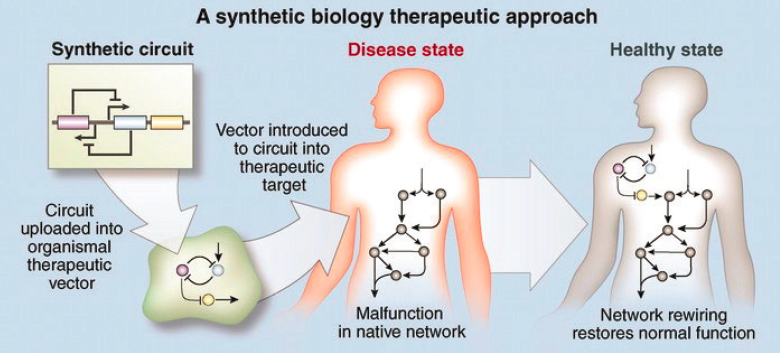
cellular morphology, and the particle size of ground corn kernels all affect ethanol yields. Ideas from biological physics have been instrumental in the development of today’s ethanol processing capabilities, and technologies including scanning electron microscopy, laser confocal microscopy, small-angle X-ray scattering, and X-ray diffraction are revealing key morphological properties of maize starch that can be exploited for further process improvements. These technologies and genome editing have also led to advanced biofuels, which are produced from non-food biomass including agricultural waste, paving a future for more sustainable approaches to energy independence.
Genetic networks are one of many types of networks that are present inside cells. Metabolic networks connect many different enzymes and turn food molecules, which the cell takes up from its environment, into fuel needed to power all the machinery of the cell. Signaling networks relay information about the cellular environment and interface with both genetic and metabolic networks. On an even larger scale, populations of single celled organisms can be controlled by manipulating the interactions between individual cells.
Efforts to engineer new functionality in genetic networks have highlighted, in several cases, our lack of understanding of the underlying basic science. While there are qualitative pictures of the relevant regulatory interactions, and quantitative models for bits and pieces of the larger networks, there is no compelling theoretical framework within which to constrain the design problem. Although it is tempting to make analogies to electronic circuit design, which reaches extraordinary heights in modern computer chips, we still are missing important ingredients: We do not have a completely reliable quantitative description of the basic molecular interactions, which play the role of device physics in semiconductor circuits, and we just scratched the surface of the functional logic that genetic circuits can implement. To fashion synthetic biology into a true engineering discipline will require understanding the quantitative relationships between the microscopic interactions of the molecular parts and the emergent, cell-scale properties of the network. Again, basic scientific questions in biological physics are linked closely to opportunities for new technologies.
PREDICTING AND CONTROLLING EVOLUTION
The evolution of microbial and viral pathogens has played a central role in human history, and each of us has experienced the impact of microbial and viral evolution on our daily lives. Human health is increasingly threatened by the rise of antibiotic resistant bacterial pathogens, and by epidemics caused by viruses such as seasonal influenza, HIV, Ebola, Zika, and most recently SARS-CoV-2. This has led to intense efforts to infer the history of these pathogens, to predict their future, and even to manipulate the environment in ways that can control and alter these
evolutionary processes. Methods originating in the biological physics community have played an important role in all of these efforts.
In recent years, widespread sequencing has improved our ability to understand and track emerging epidemics. By reconstructing phylogenies from this sequence information and overlaying them with the time and location of each sample, one can trace the transmission networks through which an epidemic spreads. One excellent example of the contribution of biological physicists to this endeavor is in the development of Nextstrain, a widely used analysis and visualization tool (see Figure 7.5). This tool has been used by researchers, journalists, policymakers, and the public to understand the spread of seasonal influenza, West Nile Virus, Zika, Ebola, and SARS-CoV-2. This has helped guide public health decisions by making it possible to evaluate the impact of policy decisions and epidemiological conditions. It also has provided important early insight into emerging threats.
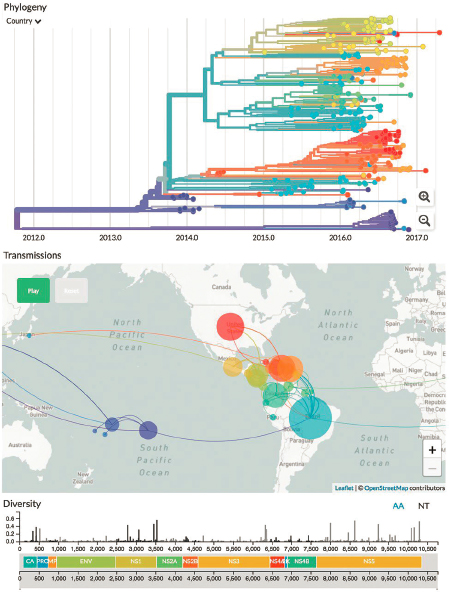
For example, one of the co-inventors of Nextstrain used these tools to show that community transmission of SARS-CoV-2 was occurring in the Seattle area in January 2020. Because these approaches are based on sequence information, they also can help us identify and track novel variants of concern based on their effects on phylogenetic structures.
Understanding microbial and viral evolution also is critical to efforts to predict these evolutionary processes. For example, rapid evolution in seasonal influenza allows the virus to evade the immune response to earlier strains. In order for vaccines to keep up with this, one must predict each spring how the virus will evolve over the following year, in order to have an appropriate vaccine available the following fall. Recent work from the biological physics community has introduced new approaches to use the statistical structure of influenza genealogies as the basis for inferring the relative fitness of different currently circulating strains, and hence to make predictions about their likely importance in the coming year.
The ability to predict evolution is also central to any attempt to control it. In the past few decades, there has been an increased appreciation for the fact that in many cases, disease treatments must consider not only their impact on a pathogen in its current form, but also how the treatment regime will influence the pathogen’s evolution. A key example comes from the treatment of HIV, where early treatment regimens typically used antiviral therapies one at a time, which often led to the sequential evolution of resistance to each therapy, followed by treatment failure and eventually death. Analysis of kinetic experiments in the clinic established that the virus was reproducing rapidly despite the long time required for the disease to manifest. Furthermore, because viral replication occurs without proofreading (Chapter 2), mutation rates are high. Theoretical work from the then nascent biological physics community showed that, combining these observations, essentially all single- and double-point mutations were accessible to the virus on time scales that matter for treatment, but triple mutations are not. This means that there are almost no paths for the virus to evolve resistance to three different drugs simultaneously. This is the origin of the three-drug cocktails that revolutionized HIV treatment, making it possible to control the virus indefinitely and saving millions of lives.
Related considerations are at play in designing treatment regimes involving other antiviral or antimicrobial therapeutics, where analyzing the pharmacokinetics of the drug within an individual patient as well as the distribution of the drug across the larger population is essential in controlling the evolution of resistance. More recent work has also begun to consider the potential to exploit interactions between drugs to design dosing regimes that can reverse or severely limit resistance.
Inferring and controlling evolutionary dynamics also plays an important role in the study of cancer biology. Since any cancer is the result of a somatic evolu-
tionary process, by using the tools of population genetics to analyze sequence variation within cancers we can make inferences about the effects of natural selection on putative driver and passenger mutations. This can shed important light onto the molecular mechanisms underlying cancer progression, and also has the potential to help in determining prognoses and in designing personalized treatment regimes based on the genotype of a particular cancer. Major investments in cancer sequencing have created enormous data sets for this purpose, and researchers from the biological physics community play an important role in designing methods, especially theoretical and computational methods, to exploit these new resources.
BIOMECHANICS AND ROBOTICS
Many people have envisioned a future where our lives are made easier by robots. In some ways, that future is now—many once dangerous and mind-numbing jobs in factories now are performed by special purpose robots. But what about robots with more general capabilities? Perhaps surprisingly for those outside the field, some of the biggest challenges are with everyday tasks, such as walking or running over complex, real world terrain. For the biological physics community, trying to understand why these problems are so hard is part of making precise what is meant by biological function, or specifying the physics problems that organisms must solve in order to survive (Chapter 1). In particular, the emerging focus on the physics of behavior (Chapter 1) creates the opportunity for deeper connections between robotics and the physics of living systems, moving beyond analogies and inspiration to testing common physical principles.
The mechanics of swimming, flying, and walking captures the imagination of scientists and the lay public alike, drawing appreciation for the physics of charismatic organisms such as water-walking insects, loping dogs, sidewinding rattlesnakes, flapping hawkmoths, and flocks of birds. The field makes connections across the vast expanse of field biology to theoretical physics, and provides numerous examples that are suited for teaching at all levels. Organisms’ biomechanical capabilities also are inspiring the exploration and development of new materials. One example is the effort to create artificial dry adhesives mimicking the properties of gecko feet. Such adhesives would be valuable for their self-cleaning properties, ability to stick underwater, and ability to be placed and removed multiple times without losing their stickiness. Research in this area has yielded technologies that now are approaching commercialization.
At some level, all robots are inspired by the phenomena of life. To the extent that they are being built of very different materials, it is clear from the outset that one must transfer system-level principles rather than microscopic mechanisms. How far can this approach be taken? Among the possibilities are microrobots
swimming through the bloodstream to fight disease; humanoid robots serving as caregivers; dog or snake-like robots searching for survivors in rubble; and self-navigating robots that drive us around or deliver our packages. There is a long and complex history, from ancient legends to successful modern factory automation. While the field is oriented toward practical applications, there is a theoretical side to robotics that is mathematically deep, drawing on differential geometry, dynamical systems, and more.
Some of the most successful recent developments are robots that incorporate mechanics into their control and morphology. These designs are based on the idea that effective locomotion in complex environments requires not only active controls but also well-chosen passive mechanical elements. This interplay emerged from studies of a number of different living systems, from cockroaches to kangaroos. Organisms tend to bounce in similar ways such that they can be described by a so-called spring loaded inverted pendulum (SLIP) template. Templates are essentially low order models that incorporate minimal dynamics displayed across groups of organisms. Dynamical systems integrating mechanics (nonlinear dynamics) and control have played an important role here in analyzing the important role of stability of such templates. An excellent example of how this interplay translates to robotics is the 6-legged robot RHex (see Figure 7.6E).
A critical idea here is that appropriate template dynamics can simplify control of movement, in essence offloading neural (and electronic) computation to the mechanics and body. This is in stark contrast to the philosophy of tightly controlled robots that have necessarily dominated robotics in factories. Indeed, the emergent aspects of living systems allow dynamics that are good in simple environments to on occasion be good in more complex terrain. Studies of cockroach response to perturbations on time scales faster than neuromuscular feedback loops coupled with SLIP directly led to RHex, which despite its simplicity displays impressive rapid running and complex environment performance. While RHex was commercialized in the 2000s, it has remained niche. However, the fruits of legged robotics work inspired by the SLIP template are now starting to appear in the real world, moving from laboratories to commercialization and deployment in real-world applications such as inspecting buildings. The doglike Spot robot (see Figure 7.6D), which takes advantage of both physical modeling and gauge theory principles, is one such example.
If walking is complicated, perhaps it would be easier to move without legs at all. Several groups in the biological physics community have focused their attention on the mechanisms of locomotion in snakes. In many cases, these organisms are moving on or through granular materials such as sand or soil, and the mechanics of such materials is itself an interesting and active physics problem. One common pattern of movement in such organisms is side-winding (see Figure 7.6C), which is quite effective over diverse terrain. Physicists study-
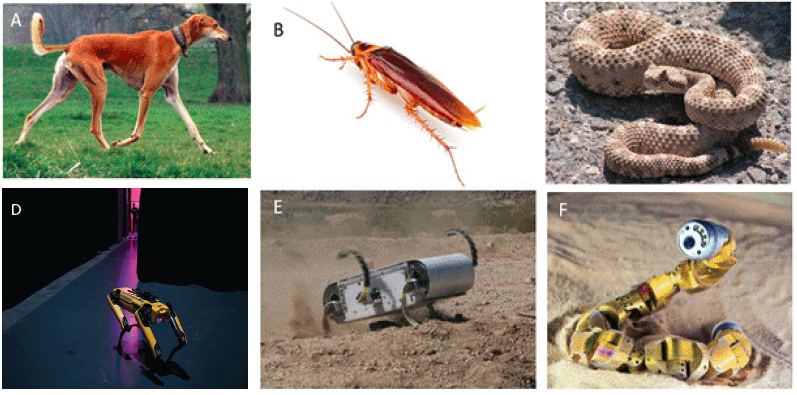
ing actual sidewinders revealed a new “two wave” template that captures the sidewinding dynamics and reveals how phasing and modulation of the waves can lead to high-performance propulsion, maneuverability and stability. This description lends itself naturally to gauge theory formulation, importing ideas from the analysis of life at low Reynolds number (Chapter 1). Implementation of these principles in limbless robots has led to significantly improved mobility, illustrating the power of interaction among traditional biomechanics, robotics, and biological physics (see Box 7.2).
Efforts in the exploding field of soft robotics will certainly aid in robophysical modeling of living systems as well as lead to advances in robots that perform in real-world environments. In fact, concrete evidence for the promise of such systems is accumulating, as illustrated by the example of a soft, fish-like robot that recently explored the ocean at a depth of 3,000 m and will soon swim in the Mariana trench at depths of 10,000 m. This device takes advantage of biological-like propulsion (flapping), and is constructed from a soft elastomeric body and fins. This example demonstrates that advances in materials, sensors, actuators, and control principles can enhance the ability to instantiate principles discovered in
living systems, enabling engineered devices to move in hydro-, aero-, and terradynamic environments that are presently challenging for human-made systems.
NEURAL NETWORKS AND ARTIFICIAL INTELLIGENCE
One of the most consistent themes in the history of technology is the idea of building machines that emulate the extraordinary functionality of living systems. Perhaps flying like birds is the most ancient example of this. Building a machine that processes information in ways that mimic the function of the brain—artificial intelligence (AI)—is a more recent, but arguably even more grand idea. There have been important successes with pieces of this problem, such as acoustic signal processing that is modeled on the mechanics of the inner ear, or decomposing complex signals into positive parts, emulating the fact that neurons cannot generate negative action potentials. But since the turn of the 21st century, there have been startling developments which bring the grand dreams into sharper focus.
Emulating the brain depends on having computing hardware, but also on having a theoretical framework that defines more precisely what the brain does and how it does it. Systematic efforts in this direction began in the 1800s, with the formalization of “laws of thought” as mathematical logic. Twentieth-century discoveries about the dynamics of individual neurons, and the nature of their connections, seeded the idea that models of neural networks could embody computational functions. As described in Chapter 3, even simple models of individual neurons, if connected in arbitrary ways, can generate complex dynamics and have considerable computational power. Progress would require simplification.
The first great simplification in neural network architecture was to focus on layered structures, so that information flows from one layer to the next without feedback. This is loosely inspired by layers of processing in the brain itself. The “perceptron” architecture appears around 1960 (see Figure 7.7), and enthusiasm would wax and wane over decades. Today, perceptrons with many layers—deep
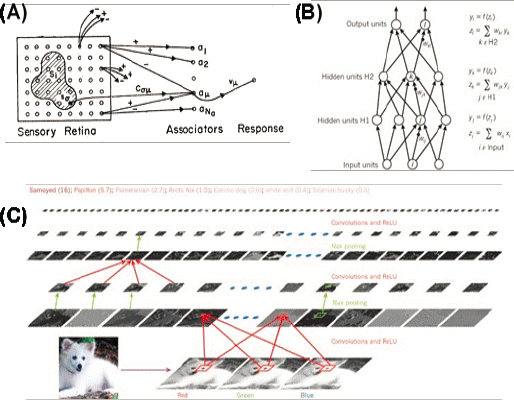
networks—are the foundation for the first machines that achieve human-level performance at tasks such as image recognition and game playing. The biological physics community and the physics community more generally have made important contributions to these remarkable developments.
Neural networks work because they are trained to perform particular tasks. The actual computations done by a particular network depend on the strengths of the connections between neurons, and a crucial part of the neural network idea is that these connections are plastic, as with real synapses in the brain. Learning rules for how synaptic strength changes can be modeled on what is known about synapses, but the engineering environment also allows going beyond the constraints of known mechanisms. This has been a productive dialogue, with more subtle forms of learning and adaption in the brain being applied to artificial systems and conversely the success of different learning rules driving re-examination of what is known about brains. More abstractly, the perceptron provided an important testing ground for theories of learning, especially for approaches grounded in statistical mechanics.
The push to develop truly deep networks was driven by classical theoretical results on the limitations of single layers, by experimental discoveries about the hierarchy of processing in the visual system, and by intuition. For image processing, there is an additional physics-based intuition that computations should be translation invariant, so that a small patch of the image will undergo the same transformations no matter where it appears. Taken together these ideas lead to convolutional networks, as in Figure 7.7C, which provided some of the first examples of human-level performance at classification and recognition of complex, real world images.
The influx of ideas from statistical physics to neural networks emphasizes that signal processing often involves an implicit model for the probability distribution of the incoming signals. This idea has been central to thinking about coding in real brains (Chapter 2) and the adaptation strategies that maintain the efficiency of these codes in varying environments (Chapter 4). In deep networks, it can be made concrete, (roughly) running the network backward to generate rather than process images. This has led to strikingly beautiful images, sparking the imagination. But it also has led to “deep fakes,” which can be difficult to distinguish from real images, even of current events.
More recently, attention has turned to networks that have feedback and hence nontrivial dynamics. These recurrent networks are especially interesting for the processing of speech and language. In just a few short years, these networks have driven a revolution in our ability to interact with machines in natural language, and to use machines as language oracles, as in machine translation. A central problem is that real language has correlations on all scales. The relation between network architecture, correlation structure, and effectiveness in language understanding is a very active area of research. This work has strong overlap with ideas from the
biological physics community about the physics of behavior and the emergence of long time scales in neural dynamics (Chapters 1 and 3).
As often is the case, the neural network revolution has drawn from basic science and is feeding back into basic science. At a practical level, deep networks are being deployed for the first steps of data reduction in many biological physics experiments. Deep networks also provide engineering solutions, of startling effectiveness, to problems ranging from protein structure prediction (Chapter 6) to the identification of genes relevant to disease. There are serious discussions about whether deep networks trained on image classification tasks, for example, provide models for the detailed responses on neurons in the visual system. More deeply, there is the problem of why these networks work: As models for the mapping of input to output, or for the dynamics of a process such language, they are enormously complex, sometimes with billions of parameters. This total number of parameters vastly exceeds the number of examples used in training the network, strongly violating our intuitions about “good” models. How is success possible in this under-determined regime? Can we make progress using the sorts of statistical physics methods that gave the first insights into neural network learning a generation ago (Chapter 4)? Does this influence thinking about the brain itself, which after all has roughly 100 trillion synapses? In specifying the problems that the brain is capable of solving, is it essential to include constraints on how much data is available from which to learn the correct solutions?
Neural networks today generally are implemented as simulations on digital computers. An alternative, which also goes back to the origins of these models in the biological physics community, is to build special purpose hardware, taking seriously the analog nature of computation in the nervous system. This is a rich field, which requires thinking about how basic mathematical operations carried out by neurons could be realized by semiconductor device physics. Among many other issues, such analog computations can be done at much lower power. This observation leads back to thinking about physical principles for brain organization, asking, for example, when it is more efficient to generate action potentials and when it is possible to compute with sub-threshold voltages. As a practical matter, special purpose “neuromorphic” chips have found application in niches where low power dissipation is especially important, starting with early touchpads and fingerprint sensors.
An important lesson from the long and complex history of neural networks and AI is that revolutionary technology can be based on ideas and principles drawn from an understanding of life, rather than on direct harnessing of life’s mechanisms or hardware. Although it may take decades, it thus is reasonable to expect that principles being discovered today will inform the technologies of tomorrow.