8
Land Use
ABSTRACT
Land used for agriculture and forestry plays a significant role in U.S. decarbonization strategies. It serves as a potential terrestrial carbon sink (in forest biomass and agricultural soils), as a major source of the greenhouse gases (GHGs) CH4 and N2O, and—through bioenergy production capacity—as a partial replacement for fossil fuels and a potential source for carbon capture and storage. In addition, land availability constrains components of decarbonization within the energy sector (e.g., wind and solar facility siting, transmission grid expansion).
The available “safe” land carbon sink capacity in the United States is more than sufficient to support the negative emissions needed from the land sector consistent with 2050 net-zero goals. Funding from the Inflation Reduction Act (IRA) to incentivize terrestrial carbon sinks and non-CO2 emission reductions is technically capable of generating an annual land sink plus CH4 and N2O abatement of 211 Mt CO2e/y in 2030, and a total net emission (carbon sinks plus non-CO2 abatement) reduction of 845 Mt CO2e over the 8-year funding cycle from the IRA, at implementation costs ≤$50/tonne CO2e. This exceeds the estimated land sector contribution needed to follow emission reduction trajectories in current comprehensive U.S. decarbonization scenarios. However, there is substantial uncertainty with respect to the extent that IRA investments will actually achieve these potential emissions reductions, and monitoring, learning, and adaptive management will be necessary for lands to play a meaningful role in decarbonization.
Land use sinks and sources of GHGs are among the most difficult to accurately quantify because they are non-point source, highly variable both spatially and temporally, and subject to many influencing factors. Hence, Recommendations 8-1, 8-3, 8-4, and 8-5 are for the Secretary of Agriculture to direct funding to improve measurement and monitoring of forest and agriculture soil carbon sinks and CH4 and N2O emissions, and to better track practice adoption rates, barriers to adoption, and overall performance of the land use–related climate mitigation initiatives in the IRA and related legislation. Demand-side factors—including development of artificial meat and dairy food products, reduced food waste, and shifts toward a more plant-based diet—could significantly reduce land needed for agricultural production. Sufficient consumer acceptance of and demand for these products (see Recommendation 8-8) could cut N2O
emissions associated with fertilizer use, reduce livestock-sourced CH4 and N2O emissions, and free up substantial land area for expanding perennial forest and grassland systems with high carbon sink capacity.
INTRODUCTION
Positive emissions of carbon dioxide (CO2) from terrestrial ecosystems to the atmosphere occur whenever photosynthetic uptake of atmospheric CO2 is smaller than emissions from plant respiration, biomass combustion, and microbial decomposition of dead biomass. Positive emissions cause an ecosystem to lose carbon mass because of a net transfer from terrestrial organic carbon to atmospheric CO2.1 Carbon dioxide emissions are net negative whenever losses are smaller than gains, which causes a net gain in ecosystem carbon stocks (a carbon sink) and a net reduction in atmospheric CO2. In addition, terrestrial ecosystems—particularly those managed for agricultural production—emit two other potent GHGs: methane (CH4, primarily from livestock production) and nitrous oxide (N2O, primarily from synthetic fertilizer). This chapter covers four kinds of GHG mitigation measures involving land—specifically, forests croplands, and pastures, all of which are targeted by the IRA2:
- Increasing negative CO2 emissions through reforestation and afforestation and changes in forest management.
- Reducing positive emissions caused by wildfire through changes in forest management.
- Increasing negative CO2 emissions by promoting agricultural practices that increase carbon stores in soils.
- Reducing CH4 and N2O emissions from agriculture and animal husbandry.
Additionally, this chapter discusses land requirements for siting energy infrastructure (e.g., wind turbines, solar panels, and transmission lines); land requirements for production of biofuels feedstocks; and the land use impacts of animal agriculture and the potential implications of demand-side changes.
The 2021 National Academies’ report Accelerating Decarbonization of the U.S. Energy System deferred discussion of the policies to create and manage agricultural and forestry carbon sinks to this report, and hence a full chapter has been allocated here. This chapter is unusual, in that many of the technical and scientific methods for achieving
___________________
1 While carbon transport occurs between land and water (rivers, oceans) in terrestrial ecosystems, this chapter will focus only on land.
2 In contrast to the IRA, neither the Infrastructure Investment and Jobs Act (IIJA) nor the CHIPS and Science Act includes programs or funding for terrestrial carbon sinks.
negative CO2 emissions in the land use sector were addressed comprehensively in the 2019 National Academies’ report Negative Emissions Technologies and Reliable Sequestration: A Research Agenda. That report covered strategies and achievable CO2 removals for (1) and (3) above as well as biofuels used for Biomass Energy with Carbon Capture and Storage (BECCS), in which biomass is used to produce electricity, hydrogen, or a carbohydrate fuel, with CO2 capture and geologic storage.3 However, mitigating agricultural emissions of non-CO2 GHGs were not covered in the 2019 report, and so here an analysis is provided—including available practices and magnitude of mitigation potential—of N2O and CH4 reductions that play a key role in any net-zero GHG emissions pathway. The committee will not recapitulate everything in the 2019 report but will summarize and update its findings about terrestrial GHG mitigation. In the 4 years since the release of the 2019 report, the most important relevant new developments are:
- Terrestrial ecosystem GHG mitigation has become a more prominent topic in the public and scientific literature under the rubric of “Nature-Based Climate Solutions” (NBCS).4 Several state governments and the federal government are giving increased emphasis on NBCS approaches (California Natural Resources Agency n.d.; White House 2022). Several comprehensive and peer-reviewed analyses of the potential of NBCS have been published, including some that caution against previous estimates that NBCS could supply more than one-third of needed climate mitigation (e.g., Cook-Patton et al. 2021a,b; Fargione et al. 2021; Seddon 2022; Seddon et al. 2020).
- There has been rapid growth in private markets for carbon offsets, many from forestry and agricultural practices (see Box 8-2 below). Some private companies have established large-scale experiments intended to verify the negative emissions they sell, like those described later in this chapter. However, these experiments are not particularly useful for safeguarding the public expenditures for carbon sinks in the IRA, because the information is proprietary. Also, concerns persist about the permanence, additionality, and leakage of carbon offsets. Permanence is a concern because a negative emission that is subsequently returned to the atmosphere, resulting in only short-term temporary storage, largely negates the intended mitigation benefit.5 Additionality means that the offset would not have occurred without the payment to adopt C sequestering practices; incentives are wasted when provided where practice
___________________
3 Note: Not all biomass is converted to electricity, and biomass removal can potentially degrade the land sink.
4 “Nature-based solutions” (NBS) is also frequently used in the same context.
5 There is still value in temporary storage that spans a few decades, as it could buy time for other more technical or costly sequestration solutions to be developed and deployed.
- changes would have occurred anyway (non-additionality). Leakage occurs when an offset in one location simply shifts the emissions to another—for example, when reduced deforestation causes forest clearance in another location because of unabated demand for new cropland and/or timber products. To accurately quantify carbon removal for a given offset, one must know how much additional carbon a project removes from the atmosphere and the magnitudes and timing of any subsequent re-emissions owing to lack of permanence.
- The market for plant-based artificial meat has grown substantially. Meat substitutes might offer the potential to significantly reduce constraints on GHG mitigation caused by limited arable land, and by competing demands for food and fiber production and biodiversity preservation. The National Academies (2019) reviewed the abundant literature showing that global managed land mainly comprises croplands, grasslands, and forests. Today’s croplands and grasslands will be needed to feed increasingly wealthy and numerous humans through midcentury and beyond, given current demand for animal protein. Thus, the land for large-scale additional deployment of land-hungry methods of carbon mitigation, such as forest planting or biofuels feedstock production, must come either from agricultural land or forest. If this land comes from agricultural land, then this could cause food price increases or shortages, which have repeatedly caused political unrest and violence in the past (Bellemare 2015; Calvin et al. 2014; Kreidenweis et al. 2016; Powell and Lenton 2012; Rosegrant 2008; Smith et al. 2013). If it comes from forests, then this will harm biodiversity, particularly in tropical forests, and release carbon currently sequestered in the forests (Law 2022). While the use of degraded agricultural lands would partially mitigate these concerns and could provide additional benefits, the National Academies (2019) were unable to identify a large body of these degraded lands. Meat substitutes have the potential to free up cropland currently devoted to animal feed production to directly feed more people or to provide services in reforestation or sequestration (Santo et al. 2020). Meat substitutes are discussed at greater depth later in this chapter.
- The National Academies (2019) reviewed the possibility of adding crushed mafic and ultramafic rocks (which are silicate minerals high in base [e.g., Mg, Ca] cations) to agricultural lands, but only as a frontier negative emissions technology. These rocks react with CO2 from the atmosphere, yielding stable carbonate minerals and providing co-benefits by increasing soil pH, which improves the productivity of acidic soils (Beerling et al. 2020). Many start-up companies have emerged to bring agricultural carbon mineralization to market, and the technology now appears much closer to deployment than it did 4 years ago.
In what follows, the committee analyzes gaps and barriers between the terrestrial carbon sinks (biomass and soils) needed for the United States to reach net-zero emissions by midcentury, and those that might be created by the IRA. Next, the committee analyzes mitigation measures in the IRA that target agricultural-based emissions of methane and nitrous oxide. The committee offers specific recommendations to the Secretary of Agriculture about the implementation of programs in the bill that target carbon sinks through forestry and agricultural soils, and through abatement of methane and nitrous oxide. The committee then addresses the total amount of land needed for the net-zero transition, including with the role of biofuels feedstock production. Last, the committee discusses the potential role of meat and dairy substitutes as a demand-side approach to reducing net GHG emissions from the agricultural sector. Table 8-3, at the end of the chapter, summarizes all the recommendations that appear in this chapter regarding land use in support of decarbonization efforts.
TERRESTRIAL CARBON SINKS NEEDED TO REACH NET-ZERO EMISSIONS AT MIDCENTURY
How large do terrestrial carbon sinks need to be for the United States to reach net-zero emissions by 2050, and for the United States to reach the Biden administration’s goal of a 50–52 percent emissions reduction from 2005 levels by 2030? These two goals are nearly the same for a linearly decreasing emissions trajectory, and are treated as equivalent in the rest of this chapter.
The ~6.0 Gt carbon dioxide equivalents (CO2e) per year of U.S. GHG emissions in 2020 was about 78 percent CO2, 12 percent methane, and 6 percent N2O (EPA 2022). Here, CO2e is calculated using 100-year global warming potentials (GWPs), which means that methane has 25 times and N2O has 298 times the radiative impact of an equivalent mass of CO2 (EPA 2022). These GWPs are from the Intergovernmental Panel on Climate Change (IPCC) AR4, which is the current convention for national reporting. Updated 100-year GWP estimates from the IPCC AR6 are 27 for CH4 and 273 for N2O.
The current U.S. emissions of 6.0 Gigatonnes CO2 equivalent per year (GtCO2e/y) do not include a net CO2 sink of 0.8 GtCO2 from the Land Use, Land-Use Change, and Forestry (LULUCF) sector, which is caused primarily by forest regrowth, probably amplified by CO2 fertilization of tree growth (EPA 2022). This sink counts as part of U.S. net anthropogenic emissions under United Nations Framework Convention on Climate Change
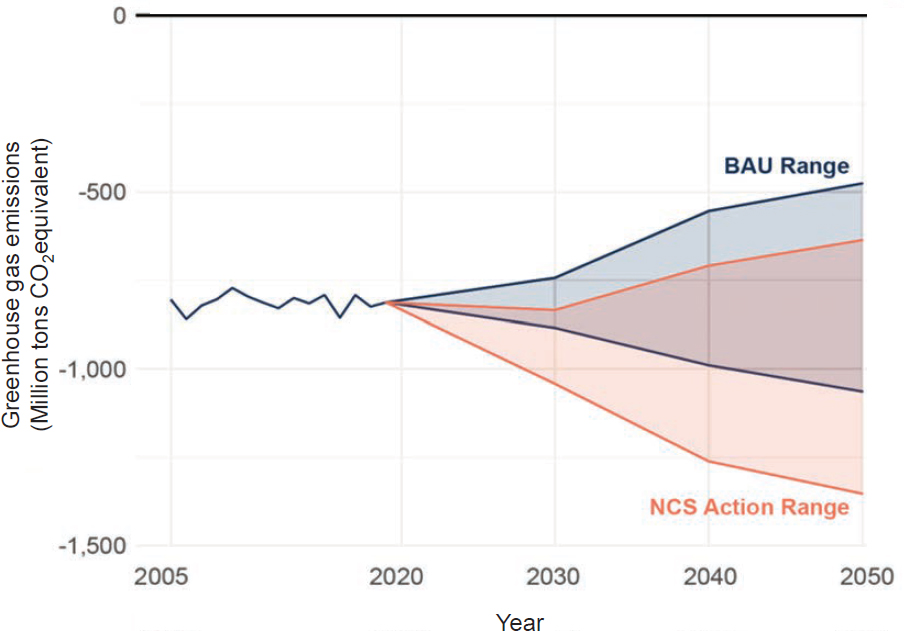
(UNFCCC) guidelines.6 The LULUCF net CO2 sink has declined from 892 Megatonnes CO2 per year (MtCO2/y) in 1990 to 812 MtCO2/y today because regrowing forests in the eastern United States are beginning to reach maturity and because of growing losses from wildfire and insect pests primarily in the west (EPA 2022). Furthermore, the current net CO2 sink from LULUCF is uncertain; EPA (2022) reports 95 percent confidence limits of 648 and 1076 MtCO2e/y. There is also considerable scientific uncertainty about the future sink because of the difficulty of balancing losses that are linked to climate change with gains owing to CO2 fertilization and recovery from historic land cover change, as well as unknown future forest harvesting, deforestation, reforestation, and afforestation (Figure 8-1). These many uncertainties are also reflected in projections from the peer-reviewed literature (Hurtt et al. 2002; USGCRP 2018).
___________________
6 See United Nations Climate Change, “Reporting of the LULUCF Sector Under the Convention,” https://unfccc.int/topics/land-use/workstreams/land-use—land-use-change-and-forestry-lulucf/reporting-of-the-lulucf-sector-by-parties-included-in-annex-i-to-the-convention.
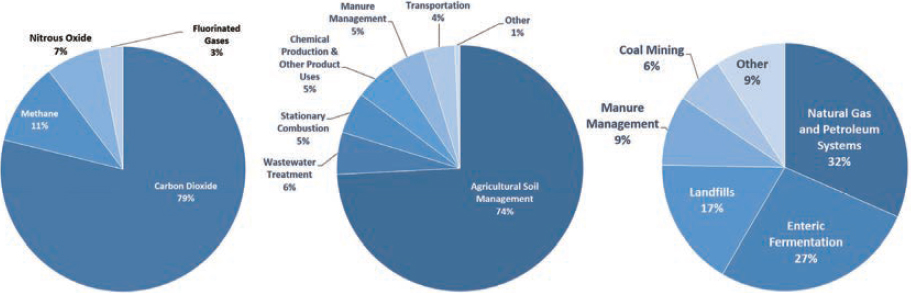
The net LULUCF GHG sink is estimated to be 759 MtCO2e because the 812 MtCO2/y net carbon sink is partially offset by CH4 and N2O emissions on forested land of 38 and 15 MtCO2e/y, respectively (EPA 2022). However, the bulk of managed land emissions of N2O and CH4 are from agriculture (which is a separate sector in national GHG inventory reporting), comprising almost 80 percent of total N2O emissions (total of 426 MtCO2e/y; 95 percent confidence range: 342–551) and 39 percent of total CH4 emissions (total of 650 MtCO2e/y; 95 percent confidence range: 596–724) (Figure 8-2).
Net-zero emissions require a CO2 sink equal in magnitude to remaining CO2e positive emissions of all GHGs covered by the UNFCCC: CO2, CH4, N2O and the fluorinated gases. Most global net-zero scenarios assume negative emissions equal to 10–20 percent of today’s emissions by the year in which net zero is achieved, which is usually 2050 in 1.5-degree scenarios (Figure 8-3). It is possible to envision extreme scenarios in which the needed sink is much smaller because of curtailed energy demand or much larger because of more residual fossil fuel use and/or higher non-CO2 GHG emissions. Large energy demand reductions like those assumed in the left-most panel in Figure 8-4 might be difficult to sustain politically in the United States, while a larger sink requirement would entail a large deployment of BECCS, like in the right-most panel, taking more land from food production and biodiversity preservation.
Most net-zero emissions budgets for the United States assume midcentury sinks totaling 1 GtCO2/y plus or minus several hundred MtCO2/y from a mix of forestry, agriculture, and industrial carbon removal methods like BECCS and direct air capture (DAC)
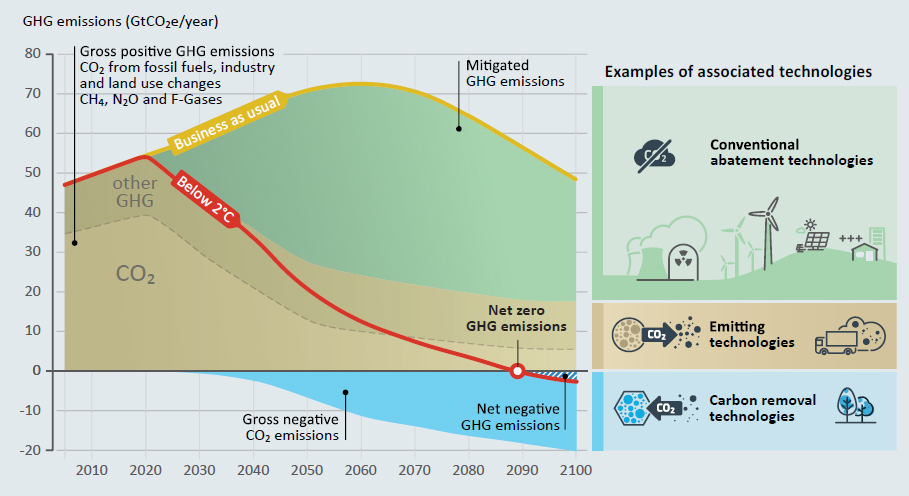
SOURCE: UNEP (2017)/Jérôme Hilaire, Mercator Research Institute on Global Commons and Climate.
(Figures 8-5 and Figure 8-6; Larson et al. 2021; Lempert et al. 2019; White House 2021; Williams et al. 2019).
Because technological options like BECCS and DAC are unlikely to be deployed at levels that would materially affect 2030 emissions, carbon sinks in the United States through 2030 should be thought of as the “business as usual” (BAU) land sink augmented by new policies designed to enhance it. In Figure 8-5, a 2030 land sink of
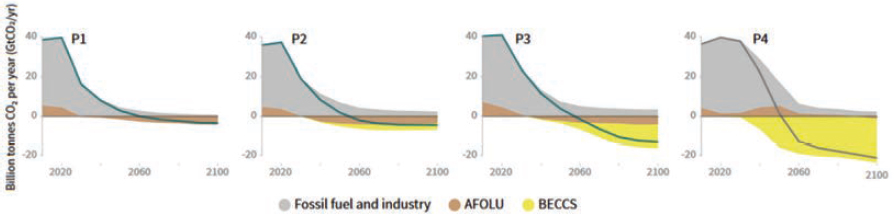
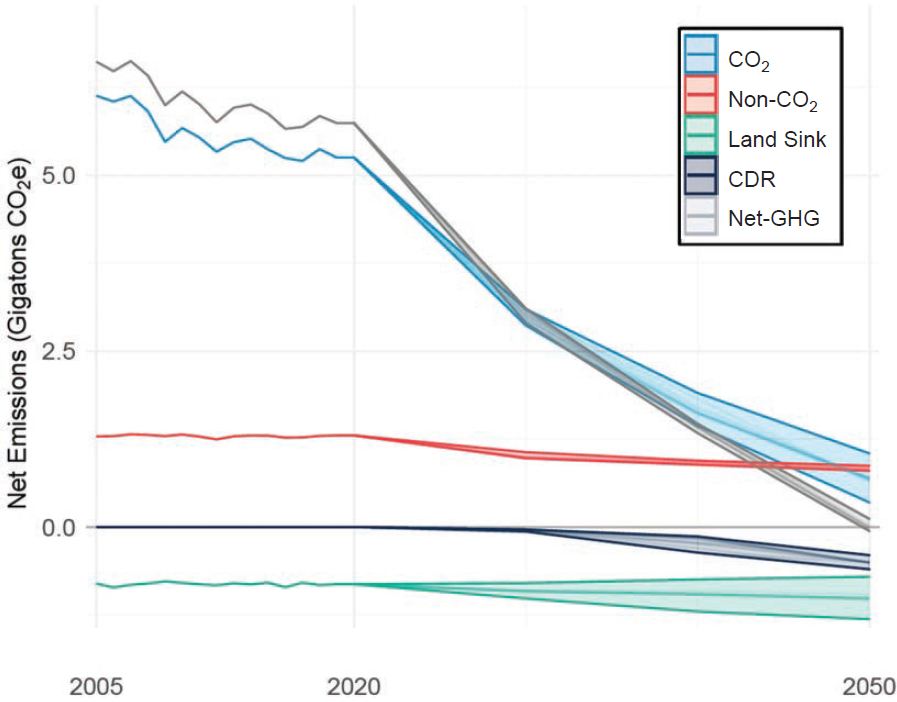
940 MtCO2/y is required to keep the United States on the assumed net-zero trajectory (defined here as the midpoint of the range shown, which is the midpoint of the “NCS Action Range” in Figure 8-1). This implies that the 2030 BAU land sink of 750–875 MtCO2/y in Figure 8-1 must increase by 65–190 MtCO2/y, with most 2030 values from other recent analyses falling within this range. For example, in Larson et al. (2021), the 2030 land sink is 750 MtCO2/y on the net-zero trajectory and 600 MtCO2/y on the BAU trajectory, which means that policies must increase the land sink by 150 MtCO2/y. Orvis and Mahajan (2021) produce a 2030 estimate of 112 MtCO2/y. The highest value that the committee has found is an increase of 336 MtCO2/y from Larsen et al. (2021), which combines optimistic assumptions about forestry and agricultural options, and assumptions about the decline of the BAU land sink. Corresponding
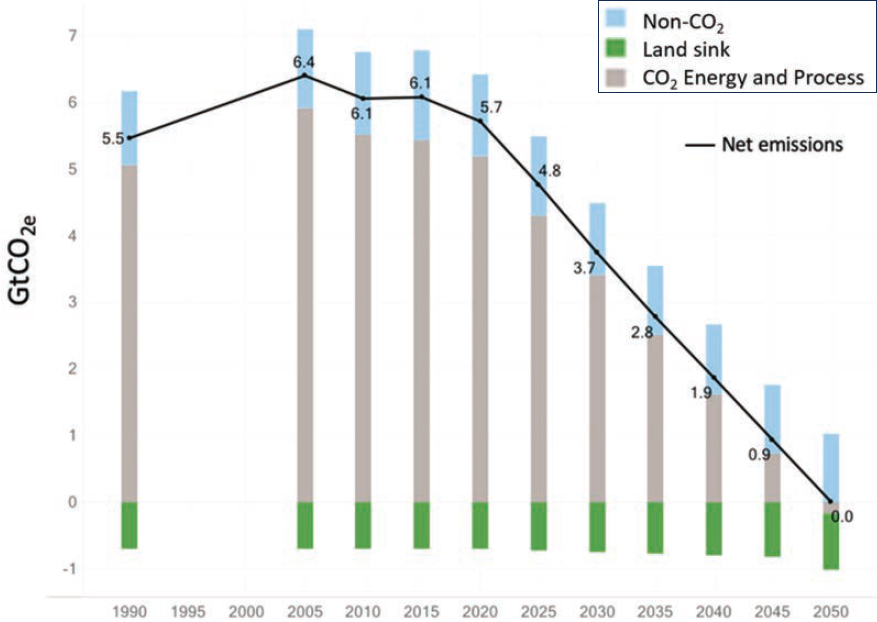
SOURCE: Larson et al. (2021), https://netzeroamerica.princeton.edu/the-report. CC BY 4.0.
numbers for new LULUCF carbon sinks needed in 2050 range from zero to 495 MtCO2/y, because of the large range of uncertainty in the BAU trajectory in Figure 8-1. In the rest of this chapter, the range from the White House strategy paper is used because it encompasses most published values and reflects current U.S. policy goals.
The IRA covers the next 8 years of the 28 years to midcentury, and so it is useful to consider a linear ramp from zero in 2023 to 65–190 MtCO2/y at the close of 2030 (sequestering an additional 9.3–27.1 MtCO2 in each year), implying a total 8-year sink of 260–760 MtCO2.
Potential Size of Safe Terrestrial Carbon Sinks in the United States
The National Academies (2019, p. 351) estimated the “safe” capacity for U.S. terrestrial negative emissions, where “safe” was defined as: no “large adverse societal, economic, and environmental impacts.” This chapter offers summary statistics about feasible and safe
land sinks. Interested readers should consult the National Academies (2019) for a detailed description of forestry and agricultural options on which these statistics are based (including rates of uptake, total capacity and cost for each option, and available U.S. land). Forestry options include avoided deforestation, afforestation, reforestation, and changes in forest management, including tree replanting after natural disturbance or fire, pesticide application, and increased harvest rotation length. Agricultural options include cropland and grazing land management practices that increase the rate of organic matter addition into soils and/or reduce the rate of carbon losses from soil. Relevant cropland practices include use of cover crops, reduced tillage, conservation buffers, perennial crops, rewetting of peat soils, and restoration of marginal lands. For grassland, improved grazing systems and/or amendments to boost plant productivity can increase soil organic carbon stocks. Application to soils of ground silicate minerals, having high base cation (Ca, Mg) content, can sequester inorganic carbon on croplands and grazing land.
The safe limit for new forests from afforestation and reforestation is primarily constrained by judgment about how much agricultural land can be converted to forest before food demand creates destabilizing price increases or deforestation elsewhere (leakage). The safe limit for sinks caused by an increased harvest interval (as a forest management option) is also constrained by the need to prevent leakage, which in this case means reducing harvest in one area can increase harvesting elsewhere to meet ongoing demand for forest products. Other forest management options, like pesticide application to reduce mortality from insect attack, have limited total capacity. The net climate benefit of afforestation can also be affected by changes in land surface reflectance (i.e., albedo) from tree planting—for example, creating a darker vegetated surface will absorb more radiation and have a warming effect that might partially offset the climate benefits from CO2 removal (NASEM 2019). In addition, all forestry options suffer from concerns about permanence, which limits opportunities for afforestation because of expected losses owing to fire and other factors on historically non-forested land (all landscapes have some degree of net carbon flux at baseline).7 Usual forest sink contracts in private markets are on the order of decades, which is longer than the 8-year duration of the IRA. This is a potential concern for permanence (White et al. 2018).
The safe limit for agricultural C sequestration is less constrained by land use change concerns compared to forestry, in that most of the management practices to increase soil carbon stocks can be employed on agricultural lands that remain in production for
___________________
7 Baselines are benchmarks that represent a one-time fixed benefit. For a meaningful market, additional carbon that is measured relative to the baseline and the absolute carbon flux into the landscape requires separate consideration. Carbon that is not sequestered permanently is at risk and should be considered lower value (Arcusa et al. 2022). See Arizona State University, “Carbon Removal Accounting Methodologies: How to Rethink the System for Negative Carbon Emissions,” https://keep.lib.asu.edu/items/170043.
food and fiber. However, some of the highest rates of soil C sequestration do involve conversion of cropland to conservation set-asides (with perennial grass or tree cover), which can result in market-based leakage if set-aside acreage is too high. Hence, use of conservation set-asides should be limited to conversion of marginal cropland, unless other co-benefits are considered to be equally important; this could result in minimal impacts on overall food and fiber production, and reduce leakage risks. In general, there is little concern with respect to leakage related to reductions in non-CO2 GHGs (i.e., N2O and CH4), in that farmers are unlikely to adopt practices to reduce emissions that would result in significantly reduced crop or livestock yields.
Safe U.S. carbon sink size limits from the National Academies (2019) are 150 MtCO2/y for afforestation/reforestation at ≤20 $/tCO2 and 100 MtCO2/y for changes in forest management that promote carbon uptake, also at ≤20 $/tCO2. For agricultural carbon sinks from improved agricultural practices, the National Academies (2019) estimated 250 MtCO2/y at ≤100 $/tCO2, if existing conservation management practices were widely deployed on agricultural lands; at least 50 Mt CO2/y is achievable at ≤20 $/tCO2 and an additional 100 MtCO2/y at 20–50 $/tCO2, based on published studies (Chambers et al. 2016; Robertson et al. 2022). These limits are also consistent with estimates in other peer-reviewed publications. For example, Fargione et al. (2018) estimated limits and costs for forestry and agricultural soils of 115 MtCO2/y, each at ≤$10/tCO2, and 520 MtCO2/y from forestry and 260 MtCO2/y from agricultural soils at ≤$50/tCO2 (not including BECCS).
Reducing non-CO2 GHG emissions lessens the size of carbon sinks required to meet overall GHG mitigation targets, and thus potential reductions in agricultural CH4 and N2O emission are relevant to include. Supporting material for a recent report by the Environmental Defense Fund and ICF of the technical potential for agricultural and forestry GHG mitigation in the United States contains marginal cost curves for reductions in CH4 and N2O emissions from agricultural sources, using current abatement technology, totaling ~90 MtCO2/y at ≤100$/tCO2e (Eagle et al. 2022).
In addition, a safe total for BECCS is 500 MtCO2/y at 20–100 $/tCO2, using only waste biomass from forestry and agriculture and feedstocks produced on lands currently devoted to corn ethanol production (NASEM 2019). Robertson et al. (2022) estimated that BECCS deployment on the 79 Mha of cropland that is marginal or abandoned or used for corn ethanol production would contribute 225–480 (mean of 349) MtCO2/y from biorefinery CCS if the land were in production for cellulosic ethanol. Assuming substitution of electric vehicles for internal combustion vehicles and full biomass utilization for CCS on the same land area would yield 370–807 (mean of 581) MtCO2/y.
Forestry and Agricultural Programs in the IRA
The agriculture and forestry programs in the IRA provide $24.5 billion to help farmers, ranchers, and forest managers adopt conservation practices that enhance landscape resilience and confer other climate benefits.
The bill directs $5 billion to forestry programs, with $2.15 billion for National Forest System restoration and fuels reduction projects (§23001), $2.2 billion for state and private forestry conservation programs (§23003), $0.55 billion for competitive grants for non-federal forest landowners (§23002), and $0.1 billion for administration (§23005). A substantial fraction of the funding is devoted to objectives other than carbon sequestration, although increased carbon sequestration could be a benefit as well. The $2.15 billion program is intended primarily to reduce the risk of wildfire. The $2.2 billion fund is divided into $0.7 billion for the acquisition of land for forest programs, and $1.5 billion for tree planting and related activities.
Tree cover, especially in urban areas and at the urban–forest interface promotes healthy living spaces. Furthermore, better land use planning, including avoiding urban development on mature forest lands and productive croplands, can reduce loss of biomass and soil carbon stocks through both direct and indirect land use change impacts (see Chapter 7). Urban reforestation can also reduce energy costs in cities through shading and evaporative cooling, as well as contributing to the forest C sink. The $1.5 billion spent on tree planting would yield a sink of 15 Mt CO2e/y, assuming the upper end of the cost estimate ($100/tCO2) for urban reforestation (Fargione et al. 2018); this could also yield energy savings for residential and business heating and cooling. Last, $0.3 billion of the $0.55 billion fund is for either climate mitigation or forest resilience. In addition, a fund of $0.1 billion for the hauling and use of material cleared for fire suppression may create a net carbon sink, but its magnitude and even its sign will depend on details of implementation. The $0.1 billion for administration is a legitimate portion of any expenditure to create carbon sinks or manage fire.
The IRA directs $19.45 billion to agricultural conservation, with the majority as additional funding to four existing U.S. Department of Agriculture (USDA) conservation programs: $8.45 billion for the Environmental Quality Incentives Program (EQIP), $3.25 billion for the Conservation Stewardship Program (CSP), $1.4 billion for USDA conservation easements (ACEP), and $4.95 billion for the Regional Conservation Partnership Program (RCPP) (§21001), with the requirement to prioritize the buildup of soil carbon stocks, the reduction of nitrogen losses and N2O emissions, and the reduction, avoidance, or capture of CH4 emissions. These programs could benefit from further assessment of environmental advantages and constraints to achieving a tighter coupling between rural
and urban waste streams and potential carbon removals, such as producing compost from food and agricultural waste and then applying as a soil amendment. The IRA also provides the USDA National Resources Conservation Service (NRCS) with $1 billion to provide conservation technical assistance, $0.3 billion to engage in a program quantifying carbon sequestration and GHG emissions in agricultural lands, and $0.1 billion for administrative expenses (§21002). It is possible that during negotiations for the 2023 or 2028 Farm Bills, the IRA funding to the programs could be rescinded and reallocated to Farm Bill baseline funding, meaning they and their climate focus get carried over more permanently in future Farm Bills. This, however, carries a risk that the rescinded money gets allocated away from climate or conservation focused programs entirely.
On top of all of this new funding, the IRA also extends the working lands conservation programs’ authorities beyond their current 2018 Farm Bill expiration in 2023 to 2031, annually at $2.025 billion for EQIP, $1 billion for CSP, $0.45 billion for ACEP, and $0.3 billion for RCPP. This, could make them more resilient in case the 2023 or 2028 Farm Bills do not pass on time, as the conservation programs would still be in place.
POTENTIAL TERRESTRIAL CARBON SINKS AND CH4 AND N2O ABATEMENT CREATED BY THE IRA
Forestry
The $2.15 billion for fire suppression may not create net carbon sinks. Current U.S. policy to reduce the risk of wildfire focuses on thinning to reduce fuel loads. Thus, there are two opposing forces. Thinning reduces forest carbon stocks, which represents a positive CO2 emission, but may reduce future fire emissions. As a result, the science is currently unsettled about the sign of the carbon flux caused by thinning treatments to reduce wildfire. Some studies show that thinning reduces forest carbon more than the reduced burning increases it (Ager et al. 2010; Campbell et al. 2012; Chiono et al. 2017; North et al. 2009), while others offer evidence that the reverse is possible (Foster et al. 2020; Hurteau and Brooks 2011).8
In contrast, all of the $2.2 billion and $0.55 billion funds target enhanced forest carbon net sinks, with the proviso that $1 billion could also be used to target related
___________________
8 Prescribed burning is another option that mimics a low-severity fire more than thinning. Prescribed burns may have fewer consequences than thinning owing to the preservation of large-diameter trees, which are often removed in unregulated thinning practices (Birdsey 2023; Hudiburg 2011; Mitchell 2012). Although there are potential benefits to prescribed burns, in some areas they create wildfire risk, especially when compounded with extreme weather.
properties such as resilience (e.g., to disaster, or severe weather). Also $100 million is directed to the Wood Innovation Grant Program (§23002), whose impact on carbon sequestration is uncertain. Investments to develop wooden replacements for structural steel and concrete in buildings is a promising option for mitigation in the buildings sector (see Chapter 7). If all of this $2.75 billion is used effectively to create net carbon sinks at $20/tCO2, then it will sequester a total of 138 MtCO2 over 8 years. A linear 8-year ramp, like the accumulating sink that would be created by planting a constant amount of new forest each year, would mean a total sink of 34 MtCO2/y in 2030. This is well below the safe total of 250 MtCO2/y from the National Academies (2019) for afforestation, reforestation, and forest management. The implication is that reluctance of landowners to enroll in the program is not the primary concern that it would be with a larger program. Only a small fraction of landowners need respond to the incentives offered by the forestry incentives in the IRA to exhaust all funds.
Agriculture
The largest proportion of the IRA funds for agriculture is devoted to increased funding to USDA programs that incentivize farmers and ranchers to adopt more conservation-oriented management practices that increase soil organic C stocks and lower N2O and CH4 emissions, as well as providing co-benefits such as reduced soil loss, greater biodiversity, and improved water quality, with its associated positive impacts on human health in rural areas. Incentives for these programs come largely in the form of cost share or direct payments for conservation practice adoption as well as technical assistance. A key issue for the longer-term efficacy of these incentives is that they be of sufficient duration to get the practice changes to “stick”—that is, to maximize continuance of the conservation management practice even if subsidies are eventually discontinued. Experience with early adopters show that it can take several years for new management practices to be “perfected” by farmers, for the improved soil and ecosystem functions to be manifested, and for increased profitability for the new practices to be sustained (Bagnall et al. 2021). Once management systems have successfully transitioned, the practice changes can be quite durable along with the increased soil carbon storage, reducing concerns over C sink permanence. Hence, incentive programs need to have sufficient duration to make it through that transition. In July 2023, USDA announced that the Biden administration will support $300 million to quantify emissions from agriculture, to include evaluating some of these new farming and soil management practices.
Currently, adoption rates of conservation practices (e.g., cover crops, nutrient management, tillage reduction, conservation buffers) are increasing, attributable in part to
recent increased government subsidies (Zhou et al. 2022). However, the rate and scale of adoption resulting from the planned increase in support payments from the IRA is uncertain. In addition to direct financial incentives, the importance of technical service providers and peer-to-peer farmer networks that can assist farmers and ranchers in adopting locally appropriate conservation practices cannot be overstated.
USDA programs, including incentives to promote conservation practice adoption, have historically underserved smaller producers, producers from Indigenous populations and communities of color, and other disadvantaged producer groups (Horst and Marion 2019). Past discriminatory practices and program design, including lack of access to finance and insufficient provision of technical services (FBLE 2022), need to be addressed to provide equitable opportunities for marginalized producers to participate in USDA programs in general, and specifically in IRA initiatives, and to contribute to improved agricultural sustainability and climate mitigation efforts.
Background on CH4 and N2O Emissions from Agriculture
In the National Academies (2019) report, forestry and agricultural carbon sinks were examined in detail, but the important non-CO2 greenhouses gases CH4 and N2O—of which the agricultural sector is a major source—were not covered. Hence, here the committee provides more background on the specific sources of these gases within agriculture, management practices to mitigate their emissions (see Box 8-1), emissions reduction potential using current technologies, and estimated costs of abatement, in order to include CH4 and N2O in the analysis of potential outcomes from the IRA and related legislation.
Methane emissions are a product of microbial metabolism in anaerobic (low oxygen) environments (e.g., the rumen and animal digestive tract, flooded soils). Agriculture is the second-largest overall emission source (behind the energy sector), accounting for nearly 40 percent of total U.S. methane emissions, mostly from livestock production, including enteric fermentation (27 percent) and manure management (9 percent), and a smaller amount (3 percent) from rice cultivation (Figure 8-2; EPA 2022). Within livestock, ruminant animals (primarily cattle and sheep) contribute the majority of emissions via enteric (digestive tract) emissions as well from the manure they produce (depending on how it is stored and managed).
Soil N2O emissions are a product of the metabolism of microorganisms present in soils, and thus they occur to some extent in all terrestrial (and aquatic) ecosystems. However, the high rates of nitrogen additions to agricultural systems used to boost plant productivity, including synthetic N fertilizers, manure additions, and N2-fixing
BOX 8 1
MANAGEMENT PRACTICES TO MITIGATE AGRICULTURAL EMISSIONS OF CH4 AND N2O
Improving feed quality, animal genetics, and health are important for decreasing CH4 emissions per unit product (meat, milk) produced. However, most livestock production in the United States is already quite efficient and there is less room for further reductions in CH4 emission intensity (emission per unit product) with conventional nutritional improvements. Thus, current research to reduce CH4 release from enteric fermentation focuses on the development of new vaccines or feed additives, such as 3-nitrooxypropan, asparagopsis (seaweed), and selective breeding of low-emission animals (Waite et al. 2022). However, these are still emerging technologies with concerns over efficacy, health, and safety. Moreover, some feed additives show initial short-term reductions in CH4 production that later attenuate as the microflora in the rumen adjust to the presence of the additive. How livestock manure is managed (largely linked to whether it is stored in an aerobic or anaerobic environment) affects both CH4 and N2O emissions from microbial processes in the waste. Various storage methods and handling can increase or decrease either CH4 or N2O emissions, but the most effective system to largely eliminate both CH4 and N2O is anaerobic digestion in which the methane is captured and then taken from a sealed reactor vessel and volatile N compounds are fully reduced to N2 gas. The biogas production also represents a renewable fuel that can substitute for fossil-derived methane, thus adding additional value to use of anaerobic digestors to manage manure. Caution should be exercised, however, if use of biodigestors only serves to enable larger concentrated animal feeding operations (CAFOs) that generate more waste. Such trade-offs require further analysis.
Reductions in N2O emissions primarily involve reducing the total amount of reactive nitrogen added to agricultural soils while increasing the nitrogen use efficiency to the plant, thus maintaining yields and also reducing other losses to the environment such as N leaching. This can be achieved by reducing nitrogen-based fertilizer and manure application through methods such as variable rate technology, using nitrogen inhibitors and “slow-release” fertilizers to suppress soil microbial activity, and improving nitrogen nutrient management through methods such as “precision farming” to optimize N efficiency (EPA 2022; Winiwarter et al. 2018).
crops (e.g., legumes), have greatly increased N2O emissions in agricultural soils compared to native ecosystems. Currently, about 74 percent of total U.S. N2O emissions are associated with agricultural soil management (Figure 8-2; EPA 2022).
Agricultural emissions of nitrous oxide and methane have been remarkably stable over the past 30 years (averaging 336 MtCO2e/y for N2O and 235 MtCO2e/y for methane), with 2020 emissions of 336 MtCO2e of N2O and 251 MtCO2e of methane (EPA n.d.(a)). Minor increases in emissions are projected by 2030, to 341 MtCO2e of N2O from agricultural soils and 265 MtCO2e of methane and N2O from livestock (EPA 2019). Methane and nitrous oxide have high GWPs, and a rapid reduction of methane emissions has been identified as the single most effective strategy to reduce warming over
the next 30 years (White House 2021). As such, agriculture presents a large opportunity to reduce emissions. However, in practice, while we can eliminate the majority of coal- and natural gas–associated methane emissions by substituting for energy from non-emitting sources (see Chapters 10 and 12), agricultural N2O and methane emissions result from natural biogeochemical processes occurring in soils and in the digestive tract of livestock that are impossible to fully eliminate and difficult to substantially reduce with current mitigation practices without affecting food production systems. Agricultural emissions will therefore come to dominate non-CO2 GHG emissions, and reducing agricultural emissions will be the major bottleneck in mitigating non-CO2 GHGs emissions. This highlights the important potential role of a dietary shift away from animal products in enabling a reduction of emissions beyond the technical methods described here (see the section “Meat and Dairy Alternatives” below).
A recent report on a 2030 mitigation pathway for U.S. agriculture identified a technically feasible 2030 target of reducing agricultural methane emissions by 63 MtCO2e/y (a 25 percent reduction from 2020) and agricultural N2O emissions by 32 MtCO2e/y (a 10 percent reduction from 2020), for a net reduction of 95 MtCO2e/y (Eagle et al. 2022), as part of a total targeted reduction of emissions from the agriculture and forestry sectors of 560 MtCO2e/y. The U.S. Long-Term Strategy uses a similar technical mitigation potential of 70 MtCO2e/y at ≤$100/tonne for livestock emissions, but just 1.7 MtCO2e/y for cropland and rice production methane and 8.8 MtCO2e/y for agricultural nitrous oxide emissions, totaling 80.5 MtCO2e/y (White House 2021).
Eagle et al. (2022) did a comprehensive meta-analysis of 16 recent peer-reviewed studies on the impact of carbon pricing on the abatement of all U.S. agricultural and forestry emissions via different approaches. They defined marginal abatement cost curves (MACCs) for each approach, which they used to identify cumulative emissions reductions per year at different prices. For compatibility with the soil carbon calculations, abatement potentials are shown for methane and nitrous oxide at ≤$20/t CO2e, at $20–$50/t CO2e, and the total at ≤$50/t CO2e, as well as the percent reduction from 2020 emissions (EPA 2022) and the total cost of said reduction (Table 8-1).
For estimating the total potential soil carbon sink plus N2O and CH4 abatement through IRA expenditures for agriculture, the committee uses the potential amounts and per tonne costs from the National Academies (2019) described above for soil carbon, and the amounts and costs from Table 8-1 for N2O and CH4 reductions. To be conservative, the abatement costs for amounts available are assumed at <$20/tonne CO2e, from the marginal abatement curves, to be priced at $20/tonne, and the costs for abatement available at $20–$50/tonne to be priced at $50/tonne. The $19.45 billion expenditure for agriculture in the IRA is more than sufficient to
TABLE 8-1 Abatement Potentials for Methane and Nitrous Oxide
At ≤$20/t CO2e | At $20–$50/t CO2e | Total at ≤$50/t CO2e | |||||||
Abatement (MtCOe/y) | Reduction from 2020 (%) | Total Cost (billions 2020 USD) | Abatement (MtCOe/y) | Reduction from 2020 (%) | Total Cost (billions 2020 USD) | Abatement (MtCOe/y) | Reduction from 2020 (%) | Total Cost (billions 2020 USD) | |
CH4 | 32 | 12.7 | 0.64 | 11 | 4.4 | 0.55 | 43 | 17.1 | 1.19 |
N2O | 13 | 3.9 | 0.26 | 6 | 1.8 | 0.30 | 19 | 5.7 | 0.56 |
Total | 45 | 7.7 | 0.9 | 17 | 2.9 | 0.85 | 62 | 10.6 | 1.75 |
NOTE: To be conservative, for cost estimates, abatement options at ≤$20/t were priced as $20/t and abatement options in the $20–$50/t class were priced at $50/t. SOURCES: Adapted from Eagle et al. (2022). Copyright © 2023 Environmental Defense Fund. Used by permission. The original material is available at https://www.edf.org/sites/default/files/documents/climate-mitigation-pathways-us-agriculture-forestry.pdf and EPA (2022).
obtain the 50 Mt CO2/y from increased soil C sinks and 45 MtCO2e/y from CH4 and N2O reductions available at <$20/tonne, which for the 8-year period of the IRA programs would total to 760 Mt CO2e (95 × 8), at a total cost of $15.2 billion (760 million × $20). This suggests that a relatively even balance of incentive funding toward C sink enhancement and toward CH4 and N2O abatement is merited for a least-cost option. The estimated opportunity for higher cost ($20–$50/tonne) C sink increases (100 MtCO2/y) and CH4+N2O abatement (17 MtCO2e/y) substantially exceeds what would remain of IRA funds after investing in the least-cost options, but that remaining funding of $4.25 billion (19.45 minus 15.2) would be sufficient for an additional 85 MtCO2e at $50/tonne, bringing the total abatement potential for the 8-year period to 845 MtCO2e. If ramped up linearly across the 8 years (like that created by annual payments for adopting cover crop or nutrient management improvements), then the annual sink and GHG reduction totals in 2030 would be 211 MtCO2/y. This is well under the “safe” CO2 removal potential that would not require changes in land use; hence, food production/food security issues would be minimally impacted in the short term and leakage owing to reduced agricultural production should not be a concern.
Adding the expected forestry and agricultural carbon sinks plus N2O and CH4 reductions, the IRA could create a net 8-year net sink of 983 MtCO2e (138 + 845) and a 2030 net sink of 245 MtCO2e/y (34 + 211, assuming a linear ramp) if available funding were effectively deployed at ≤$50/tCO2e. These are larger than the new sinks needed for the nation to stay on a linear net-zero trajectory: 260–760 MtCO2 for the 8-year total and 65–190 MtCO2/y in 2030. These estimates are consistent with the one previously published estimate for which the source calculations were available to the committee (Mahajan et al. 2022; 95 MtCO2/y in 2030).
Last, the upper bound of the 0–495 MtCO2/y range for the 2050 LULUCF carbon sink, from the U.S. National Climate Strategy analysis shown in Figure 8-1 (i.e., from the midpoint of the BAU range to the upper and lower limits of the NCS Action Range) is very close to the 500 MtCO2/y safe and practically achievable limit reported in the National Academies’ report (2019). Box 8-2 expands on how the markets for forest and agricultural carbon have developed thus far and how they may progress.
In addition to the IRA funding, it is also expected that the USDA Partnerships for Climate-Smart Commodities projects,9 currently funded at more than $3.1 billion, will produce learnings about cost-effective methods to reduce methane and nitrous oxide emissions, including improved nutrient management, enhanced efficiency fertilizers,
___________________
9 See U.S. Department of Agriculture, “Partnerships for Climate-Smart Commodities,” https://www.usda.gov/climate-solutions/climate-smart-commodities.
BOX 8-2
DEVELOPMENT AND TRAJECTORY OF FOREST AND AGRICULTURE CARBON MARKETS
Forestry and agriculture GHG mitigation projects are predominantly associated with voluntary markets,a in which buyers (e.g., companies seeking to offset emissions) purchase units of CO2 removals to (biomass or soil) sinks and/or non-CO2 GHG reductions, from projects in which farmers/foresters adopt new management practices that increase C sinks and/or reduce GHG emissions. Currently, there are very few agriculture and forestry project types that are eligible for inclusion in compliance (cap-and-trade) marketsb (e.g., California and the Regional Greenhouse Gas Initiative [RGGI] in the United States).
Recent rapid growthc in the use of offsets has been driven by companies pursuing “net zero”/“net negative” emissions policies—in particular, to offset their Scope 3 emissions.d Voluntary markets include both registry-certified offsets, with project-specific accounting protocols that account, to varying degrees, for the effects of leakage, additionality, uncertainty, and permanence on the actual abatement achieved, and climate change risks compound uncertainty (Novick 2022; Wu 2023). However, a substantial proportion of voluntary market activity involves direct transactions between buying and selling parties, without registry-certified protocols, and likely less rigor in inventory and accounting procedures. Furthermore, even for carbon market participants that base their projects on registry-certified protocols and measurement and monitoring methodologies, there is no formal standardization across different protocols, which reduces confidence in integrity of the claimed carbon removals (or GHG reductions) and opens the opportunity for selection bias for which protocols project developers might choose.
The rationale for promoting a vigorous voluntary carbon market within the land sector is that adoption of different practices that increase CO2 removals/emissions reductions incur additional costs and risks to forest and agricultural producers (e.g., delayed forest harvest, added costs for cover crop seed and management on cropland, etc.) that are a key barrier to practice adoption, which revenues from offset sales can help to overcome. Within the IRA, the major funding to forestry and agriculture is through increases in USDA programs to incentivize mitigation practice adoption through direct payments and cost-sharing. However, funding that is available to finance expanded C stock and GHG emission inventory methods and on-farm soil monitoring has the capacity to substantially improve quantification methods and reduce uncertainty in quantifying net CO2 removals. Moreover, the proposed investments in monitoring systems in the IRA will provide ongoing data on the durability of practice adoption and on practice outcomes, which is critical in addressing permanence and leakage issues particularly in the agriculture sector. Improved carbon and GHG accounting with reduced uncertainties would increase offset buyer confidence and reduce the size of uncertainty “discounts” in offset value that are widely applied by registries, increasing the value of the offset for both buyers and sellers. The expanded inventory and monitoring capabilities recommended in this chapter would contribute significantly toward developing a common set of standards for offset accounting that could allow for
harmonization across different registry systems and offset market participants. More immediately, increased transparency in the tracking and reporting of sources and protocols for offset use by the private sector can encourage these developments. (See the Chapter 11 discussion on strengthening corporate climate disclosures.)
__________________
a Prominent registries that support voluntary markets include Verra Registry, Climate Action Reserve (CAR), American Carbon Registry (ACR), the Gold Standard, and others (Stubbs et al. 2021).
b For compliance cap-and-trade markets (e.g., California and RGGI in the United States), some forest practices are allowed to generate CO2 offsets, whereas only CH4 abatement practices (from manure management and rice) are currently included as agricultural-based options.
c Globally, annual offset issuances in voluntary markets (including all energy and emission sectors) grew sixfold (46 MtCO2e in 2017 to 239 MtCO2e for the first 8 months of 2021), with forestry and land use making up 45 percent of the total market volume in 2021 (Ecosystem Marketplace 2021).
d Scope 3 emissions are emissions that are outside the direct control of a company, resulting from the use/consumption of the company’s product.
manure management, feed management to reduce enteric emissions, and soil amendments like biochar. These learnings would then need to be promoted through current USDA conservation programs, or through new policies or laws, to be broadly applied and result in emissions reductions at scale.
Forestry Carbon Sink Findings and Recommendations
Finding 8-1: The $5 billion of forestry funding in the IRA is theoretically sufficient to create additional terrestrial carbon sinks as large as those in simple net-zero trajectories. There is sufficient land available to stay well within the safe limits proposed by the National Academies (2019), and to avoid likely impediments caused by low landowner adoption rates. However, the actual performance of the terrestrial sink provisions in the IRA is still highly uncertain for several reasons, including unpredictable climate, demand for fuel and food, and changes in afforestation, reforestation, and development.
The size of the terrestrial sink needed to reach net zero is uncertain by more than a factor of 2 because of uncertainty about both the magnitude of residual emissions that will need to be offset and the fate of the current (BAU) U.S. forest sink. Moreover, the realized price per tonne of CO2 that will be sequestered by the constellation of programs in the IRA is also highly uncertain.
Afforestation, reforestation, and reduced development-driven deforestation are the options most certain to create large net carbon sinks locally. While afforestation and reforestation may promote leakage, this is less likely if marginal land is converted to forest. Also, the relatively modest size of the $2.75 billion in funding that could
promote these options reduces the danger of a reduction in agricultural land that would promote leakage. Changes in forest management such as increasing the length of the interharvest interval may create local sinks. However, an important recent paper used remote sensing methods to compare 37 forest management projects in California designed to enhance carbon sinks with matched controls and found no additional carbon storage (Coffield et al. 2022). Additionally, longer harvest rotations may reduce wood supply and increase incentives for additional harvest elsewhere.
A substantial fraction of the funding in the IRA that could promote carbon storage can also be directed toward other ecosystem benefits like “resilience.” Moreover, the IRA does not specify how landowners will be compensated or the length of contracts. Its funds must be spent within 8 years, which is shorter than the 20 years or more in most forestry offset contracts. These factors add to the uncertainty about how much carbon the bill will cause to be sequestered.
For these reasons, the committee has two recommendations for the Secretary of Agriculture, who is tasked with implementing the forestry provisions in the IRA.
Recommendation 8-1: Convene an Expert Group to Recommend Ways to Measure Additional Forest Sinks. Using the $100 million in administrative funding in the Inflation Reduction Act (IRA) for forestry efforts, the Secretary of Agriculture should convene an expert group of foresters, ecologists, and accounting experts to recommend a way to measure the sizes of forest sinks created by the IRA funding and how they change over time. Their recommendations should then be implemented. Most important is to establish appropriate control areas for a statistically representative sample of treatment areas within the first 2 years. Treatment and control areas should be resurveyed 5 years later. This, together with the Forest Inventory and Analysis census, should inform any needed revisions to management recommendations for increasing the U.S. forest sink beyond 2030 (pending subsequent legislation).
Recommendation 8-2: Prioritize Ecosystem-Level Carbon Storage. The Secretary of Agriculture should prioritize increased ecosystem-level carbon storage when allocating the $2.75 billion of non-fire-suppression funding in the Inflation Reduction Act, and especially reduced gross deforestation at the urban–forest interface, as well as increased reforestation and afforestation. When sites are selected for reforestation and afforestation, priority should be given to productive but agriculturally marginal land and degraded land, which will reduce the potential to cause deforestation elsewhere; lands with a high risk of non-permanence should be avoided. A committee of experts should review new expenditures annually to evaluate effectiveness.
Agricultural Carbon Sink and CH4 and N2O Findings and Recommendations
Finding 8-2: If allocated efficiently, the $19.5 billion of funding in the IRA for agricultural conservation directed at GHG mitigation appears to be adequate to provide the needed contribution by 2030 from soil C sinks on cropland and grazing land and from reduced agricultural CH4 and N2O emissions, for the net-zero trajectories described above. However, the actual performance of proposed measures for soil C sink enhancement and GHG abatement is uncertain for a number of reasons, and the amount of agricultural methane and nitrous oxide emissions that the IRA will avoid is highly uncertain and depends on priorities for which conservation practices are incentivized, the level of farmer/rancher participation and practice adoption, and the actual cost and efficacy of emission reduction practices. Additional soil C sink and GHG abatement could come from transitioning grain-based biofuels to cellulosic feedstocks from perennial biomass plantings. There is sufficient land available upon which to adopt new practices, and a number of farmers are successfully transitioning to more regenerative/conservation practices.
Improved monitoring and GHG inventory capabilities will be crucial to track adoption rates and durability of carbon sequestering/GHG reducing practices and whether the projected C sinks and N2O and CH4 abatement are achieved. Different management practices to mitigate agricultural emissions of CH4 and N2O vary in their effectiveness. Similarly, the efficacy of different conservation practices to store C and achievable per area rates varies by climate and soil type, the baseline management system, and other local factors. Thus, the results from providing subsidies to incentivize agricultural producers to adopt new practices will depend highly on the geographic distribution of the land area involved and the types of incentives. Additionally, rates and uncertainty bounds of soil C accrual for some practices in certain systems and geographies are poorly known. Last, the long-term durability of practices change is key to ensuring long-term maintenance of increased soil C stocks. Better understanding of the economic sustainability of regenerative/conservation agricultural practices is needed, along with improved outreach and training to assist farmers and ranchers in successfully adopting regionally tailored technologies.
The committee has four recommendations for the Secretary of Agriculture, who is tasked with implementing these programs.
Recommendation 8-3: Establish a Permanent, National-Scale, High-Quality Soil Monitoring Network. The U.S. Department of Agriculture (USDA) should establish a permanent, national-scale, high-quality soil monitoring network, as an augmentation of the National Resources Inventory (NRI) system
maintained by USDA. This would allow for periodic (re)measurement of soil C stocks and soil heath indicators within a subset (e.g., 10,000–20,000 points) of the 400,000 NRI points on U.S. agricultural lands. The current NRI system collects valuable data on land use and management practices at these locations, but does not include the on-site soil measurements that are needed for a robust monitoring program. This system would be analogous in function to the Forest Inventory and Analysis system for the nation’s forests, which entails periodic measurement of forest biomass and soils, carbon, and forest condition. Measurements of soil carbon stocks and other key soil indications at agricultural NRI points would reflect actual on-farm conditions, and could be done on a rotating basis (e.g., 1,000 points per year, on a 10-year remeasurement cycle), at low cost (e.g., $5 million–$10 million per year), and would provide the single most valuable data source to improve accuracy and reduce uncertainty in regional to national-scale quantification of soil C stocks.
Recommendation 8-4: Build Out Long-Term Agricultural Field Experiments. The U.S. Department of Agriculture (USDA) should provide funding to further build out the USDA-funded network of agroecosystem sites at land grant universities and USDA Agricultural Research Service locations, or similar long-term field experiments, to fill the gap in the existing long-term experiment site network to include agroecosystems in underrepresented cropping systems, regions, climates, and soil types, and to expand the measurement of non-CO2 greenhouse gases (GHGs), particularly soil N2O and enteric CH4 emissions on both existing and new sites. These field sites are designed to quantify impacts of adopting conservation/regenerative management practices and contrasting their performance to conventional systems. The expanded network would have a coordinated set of measurement protocols and methods, including whole-system carbon balance, utilizing eddy covariance instrumentation, and other GHG (e.g., N2O and CH4) flux measurements. The data provided would be of great value in improving capabilities to accurately quantify decarbonization and GHG emission reduction capabilities on the nation’s agricultural lands.
Recommendation 8-5: Fund Research to Quantify Indicators That Influence Adoption of Regenerative Agriculture Practices. The U.S. Department of Agriculture should fund research and survey efforts to quantify economic cost/benefit, social barriers, and equity issues influencing adoption of conservation or regenerative agricultural management practices. Considerable anecdotal evidence suggests that there are reduced input costs and higher
net returns for many farmers adopting conservation/regenerative practices. However, broader and more targeted economic and behavioral analysis is needed to project the potential for up-scaling of soil-based carbon removal and greenhouse gas reduction approaches as climate mitigation strategies. In addition, economic and behavioral research need to investigate approaches to improve policy design and address inequities in participation of disadvantaged communities in conservation incentive programs.
Recommendation 8-6: Incentivize the Abatement of CH4 and N2O Emissions and Improve Soil Carbon Sequestration. The U.S. Department of Agriculture (USDA) should split the $19.45 billion in Inflation Reduction Act agricultural conservation funding between incentive payments to abate CH4 and N2O emissions and incentive payments to improve soil C sequestration. Incentive payments should also address inequities in participation by disadvantaged communities, in line with stated USDA policies for expanding the delivery of conservation assistance to low-income and marginalized racial/ethnic communities. To maximize climate and environmental benefits, incentives should target performance (and not strictly acres enrolled) and prioritize practices that can be adopted at scale and that have the potential to continue longer term, by improving soil health, improving yield stability, and reducing input costs. Additionally, practices should be encouraged that can achieve both C sequestration and reduced CH4 and N2O emissions, as well as improve other environmental co-benefits. Such practices include conservation buffers, within field set-asides of unprofitable “patches” and precision nitrogen management.
Recommendation 8-3 could be supported under the $300 million funding in the IRA that specifically targets improvement in carbon and GHG inventory on managed lands, and Recommendation 8-4 could also be supported through the $300 million GHG inventory funds and/or via USDA’s National Institute of Food and Agriculture (NIFA) funding that supports current agroecosystem sites. Funding for economic and behavioral studies in elucidating constraints and outcomes for adoption of regenerative agricultural management practices in Recommendation 8-5 could be prioritized within current funding of USDA’s Economic Research Service and in research funding through USDA’s NIFA and possibly be included in other land use related funding lines in the IRA.
LAND REQUIREMENTS
The 915 million hectares (Mha) in the United States (765 Mha outside Alaska and Hawaii) is divided primarily among grazing lands (265 Mha), cropland (159 Mha), and forests (256 Mha), with the rest amounting to only a few percent outside developed
land and Alaskan taiga woodlands and tundra (USDA 2017). This shows why large-scale reforestation or afforestation would have to come from food-producing lands and would thus bring with it the dual risks of destabilizing food price shocks abroad and increasing deforestation elsewhere to meet ongoing demand for food (leakage) (NASEM 2019; White House 2021). Similarly, any significant increase in biofuels feedstock production would incur these same risks because it must come at the expense of lands currently devoted to food or forest products. Moreover, clearing forest to produce biofuels feedstocks would cause an initial emission in the United States of approximately 100–300 tCO2/ha from the decomposition or combustion of forest trees (Fargione et al. 2008; Hoover 2021; Law 2018). This “carbon debt” would require decades to be repaid by the production of new biofuels that displace fossil fuels, implying decades of net positive emissions, which are not congruent with a 30-year approach to net zero.
For these reasons, this report follows the recommendation of the National Academies (2019) to limit (1) biofuels feedstocks to crop and forestry residues, biomass waste, and marginal cropland and croplands already devoted to biofuels (16 Mha of corn devoted to grain-based ethanol production); and (2) changes in forest area to a few percent of the total. As benchmarks, from 1977 to 2012, U.S. forests increased in area by approximately 10 Mha (USDA 2014), which is about 3 percent of the current U.S. forest total; similarly, croplands currently kept out of production by the Conservation Reserve Program total approximately 10 Mha, which is about 6 percent of the U.S. cropland area.
With biofuels restricted in this way, the large new land use areas needed for the energy transition are for renewable electricity infrastructure and new forest carbon sinks (reforestation and afforestation). Sinks from changes in forest or agricultural management do not require land use change. Engineered sinks, such as direct air capture (DAC) would require large amounts of land, but this is a problem for the final part of the energy transition, if DAC becomes cheap enough to deploy at scale. And if it is deployed at scale, most of the land required for a DAC installation could still be used for agriculture, just like in wind farms on agricultural land. To first order then, the challenge during the next 30 years is to site all the needed wind and solar electricity and carbon sinks, while retaining the nation’s capacity to produce essential food and fiber and to preserve forest biodiversity.
Terrestrial Carbon Sinks
Technically achievable “safe” totals from the National Academies (2019) are again 150 MtCO2/y from new forests, 100 MtCO2/y from changed forest management, 250 MtCO2/y from agricultural soils and 500 MtCO2/y from BECCS. Using the midpoint
of the range of new forest sequestration rates from this study (2.6–23.5 tCO2/ha/y), attaining the “safe” upper bound for afforestation/deforestation would require conversion of 11.5 Mha of agricultural land, which is 2.7 percent of the total area (of cropland plus grazing land). Changed forest management would be needed on 70–90 Mha of existing forest lands (NASEM 2019).
Renewable Electricity
There are a significant number of studies on the land required for the wind turbines, solar panels, transmission lines, and support infrastructure of a net-zero energy system in the United States. An excellent entry to this vast literature is Saunders (2020): Land Use Requirements of Solar and Wind Power Generation: Understanding a Decade of Academic Research. Peer-reviewed studies virtually all conclude that the United States (Jacobson et al. 2015; Li et al. 2018) and most other countries (Calvert 2018; Capellán-Pérez et al. 2017; Jacobson and Delucchi 2011) have sufficient suitable land for renewable electricity infrastructure, including supporting roads and other equipment, even with the complex existing legal restrictions in the United States at federal, state, and local levels. Published studies also generally conclude that public resistance to new renewable infrastructure will significantly limit the pace of deployment and represents the most serious impediment to a 30-year energy transition (Saunders 2020). For example, only one-ninth of new renewables projects currently reach completion in the United States, after an average completion time of 5–8 years (Jenkins et al. 2021). These statistics are inconsistent with the pace of deployment needed in net-zero scenarios. Chapters 5 and 6 itemize barriers to renewable infrastructure and offers policy recommendations to speed deployment.
The most complete and fine-grained analysis of the land required by renewable electricity and transmission is offered by the Net-Zero America study (Larson et al. 2021), which planned a half-dozen net-zero electricity generation and transmission systems on a 4 × 4 km grid over the United States, using constrained least-cost algorithms. Deployment was limited by two different sets of constraints, including more than 50 factors such as high population density, legal prohibitions, Native American jurisdiction, high conservation value, low site suitability (i.e., wetlands, too steep, too little wind), incompatible prior infrastructure (i.e., airports), high agricultural productivity, and high to moderate sensitivity under the Bureau of Land Management’s designation system (Figure 8-7). The net-zero energy systems ranged from one with 100 percent renewable electricity, through a least-cost system allowing nuclear and fossil with CCS, and to a system that limited the pace of renewables deployment to the maximum historic rate. The study used National Renewable Energy Lab estimates
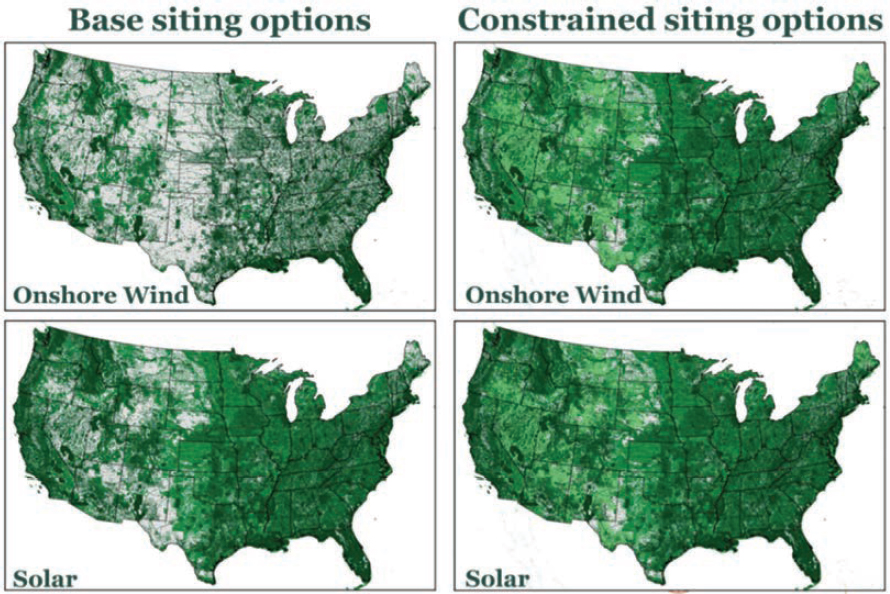
of average power density: 45 MW/km2 for solar, and 2.7 MW/km2 for onshore wind. It separately estimated the land area that would be unavailable for joint use (i.e., the area actually occupied by the wind turbines and associated access roads) and the area that could also be used for other purposes (i.e., croplands beneath a windfarm), under the assumption that the visual transformation of the landscape is the most likely source of public resistance to deployment. The study found that there is technically enough land for any of the scenarios considered, including the one with 100 percent renewables (Table 8-2), although that scenario required 90 percent of available land in the case with the strongest constraints on deployment. The large amount of visually transformed land from wind and solar in 2050—58.9 Mha for the cheapest scenario and 105.9 for 100 percent renewables—indicates a large potential for public resistance to deployment, as discussed extensively in Chapter 5.
Increased solar and wind deployment will create conflicts in land use, conservation acts, and land ownership status, affecting local communities. Promoting and
TABLE 8-2 Land Use Scenarios in NZAP
Scenario | Source | Year | GW | Million Hectares Direct Use | Mha Visually Transformed | Percent of Available Base (Stronger Constraints) |
---|---|---|---|---|---|---|
Cheapest | Wind | 2030 | 414 | 0.2 | 15.7 | 4 (14) |
Cheapest | Wind | 2050 | 1,479 | 0.6 | 55.1 | 12 (45) |
Cheapest | Solar | 2030 | 320 | 0.7 | 0.8 | <1 (1) |
Cheapest | Solar | 2050 | 1,495 | 3.5 | 3.8 | 1 (4) |
100% Renewable | Wind | 2030 | 462 | 1.7 | 17.4 | 4 (16) |
100% Renewable | Wind | 2050 | 2,700 | 10.0 | 100.3 | 22 (90) |
100% Renewable | Solar | 2030 | 402 | 2.5 | 2.5 | <1 (1) |
100% Renewable | Solar | 2050 | 2,750 | 5.6 | 5.6 | 2 (6) |
SOURCE: Larson et al. (2020), https://netzeroamerica.princeton.edu/img/Princeton_NZA_Interim_Report_15_Dec_2020_FINAL.pdf. CC BY 4.0.
incentivizing dual use of land for distributed energy resource (DER) infrastructure can help to utilize formerly contaminated areas or existing infrastructures, reducing the extra burden of construction, and can even economically revitalize communities. This can be implemented through various approaches such as “Solar pollinator”—for example, Oregon Community Solar Program (Oregon Solar 1 Storage Industries Association n.d.); “Agrivoltaics” (Fraunhofer Institute for Solar Energy Systems ISE 2020); “Floatovoltaics” (YSG Solar 2022); “Canal top” (DOI 2016); “Bridge-top”—for example, Blackfriars Bridge, London (Thameslink Programme n.d.); and many more.
Required land for electrical transmission lines is reviewed in Chapter 6. In the NZAP interim report, this was zero because the study restricted transmission to existing rights-of-way (Larson et al. 2020).
Biofuels Feedstocks
Biofuel production in the United States currently consists of approximately 15 billion gallons per year of corn ethanol, which is about 10 percent of gasoline consumption, and 2.5 billion gallons of biodiesel (including renewable diesel), which is about 5 percent of diesel consumption (USDA 2023). Corn ethanol was once subsidized but is now supported by a 15-billion-gallon annual mandate (Energy Policy Act of 2005, P.L. 109-58; Energy Independence and Security Act of 2007, P.L. 110-140), while biodiesel is supported by a $1/gallon tax credit (DOE n.d.(a)). Corn ethanol production currently requires fossil energy and yields substantial N2O emissions from fertilizer
use, such that ethanol from corn grain produces 56 percent the emissions of gasoline per unit energy (Scully et al. 2021). Biodiesel is more greenhouse efficient, with 74 percent lower emissions than conventional diesel (DOE n.d.(b)). Biofuels are marginally more expensive than fossil fuels in most locations, but within the historic range of fossil prices (DOE 2022a).
Advanced cellulosic fuels, which use most of a plant’s biomass instead of just the starches and sugars in corn kernels, can have emissions as low as 10–15 percent of fossil fuels (DOE n.d.(c); Rinke Dias De Souza et al. 2021). U.S. production of these advanced “cellulosic” fuels is only about 10 million gallons per year (EIA 2018). Such cellulosic fuels can also be produced from high-yield perennial crops like switchgrass or Miscanthus and from annual crop residues (Schmer 2008). The amount of biofuel that can be produced from an acre varies considerably depending on the crop—the ability to utilize cellulose could raise the per-acre yields from ~100–600 gallons to more than 1,000 gallons.
Perennial bioenergy crops provide co-benefits including enhanced nitrogen and water use efficiency, and some studies have reported soil carbon sequestration of 0.5–1.0 tCO2/ha/y (Glover et al. 2010; NASEM 2019). Perennials also offer the highest yields. A hectare of corn grain ethanol produces about 3,000 liters of fuel (~3,000 l/ha/y, Achinas et al. 2019), whereas advanced biofuels can have considerably higher yields—perhaps up to 10,000 l/ha/y (Coyle 2007).
As discussed in the previous section, biofuels are constrained by available land and are assumed in this report to be limited to feedstocks from biomass waste and feedstocks produced on the 16 million hectares (DOE 2022b) currently used for corn ethanol. Note that at the upper limit of cellulosic fuel yields, these lands could produce 40 billion gallons per year, which is over twice U.S. aviation fuel consumption (EIA 2019).
Biomass energy with carbon capture and storage (BECCS) can produce electricity or fuels with negative emissions (NASEM 2019). When used to produce hydrogen, BECCS provides (1) a source of industrial heat; (2) hydrogen transport fuels for fuel cell–powered heavy trucks or perhaps for aviation; and (3) a carbon sink. The combined greenhouse benefit of a carbon sink and a zero-carbon fuel might approach double that from direct biofuels combustion (NASEM 2019). With yields of 10–20 tons of perennial biomass per hectare per year on the 16 million hectares currently devoted to corn ethanol and 90 percent CO2 capture, BECCS would produce a carbon sink of 264–528 tCO2/y (assuming half the biomass is carbon). The National Academies (2019) estimated an upper bound of 500 MtCO2/y for the practically achievable U.S. carbon sink that could be produced using only biomass waste in BECCS. Thus, the upper bound including both biomass waste and dedicated energy crops on current corn
ethanol lands ranges from 0.75 GtCO2/y to just over 1 GtCO2/y. This implies that BECCS alone might fill the need for carbon dioxide removal from industrial (e.g., BECCS, DAC) and other non-LULUCF methods (White House 2022) (gray CDR range center in Figure 8-5, which has a midpoint of about 500 MtCO2/y).
Before electric land transport became economically competitive with vehicles powered by internal combustion engines, biofuels were seen as a primary means of decarbonizing road vehicle transport (Pacala and Socolow 2004). However, biofuels are now seen primarily as an energy source for low-carbon aviation and other sectors that require the high energy density of hydrocarbons. Most current net-zero scenarios assume little emissions reduction from biofuels before 2030 (Larsen et al. 2021; Larson et al. 2021; White House 2021) and heavy deployment of BECCS in the 2030s and 2040s (IPCC 2022). The optimization algorithms in NZAP (Larson et al. 2021) would deploy substantial BECCS hydrogen in the 2040s because the energy economy requires larger carbon sinks than are available from agricultural soils or forestry to reach net-zero emissions by 2050, and also needs zero-carbon fuels. As in this report, most scenarios in NZAP limited biomass production to waste and corn ethanol land, which caps available biomass at 0.7 Gt/year. Demand for BECCS was high enough by 2050 in NZAP to consume all 0.7 Gt/year, and to consume the 1.3 Gt/year allowed in the study’s high biomass scenario. This underscores the risk of an unconstrained rush to biomass that might disrupt food production and biodiversity.
Because hydrocarbon biofuels are burned in internal combustion engines or turbines and must be produced in chemicals plants, and because the agricultural production of crops for biofuels itself causes air pollution that results in approximately 2,000 air quality–related deaths in the United States each year (Domingo et al. 2021), these fuels entail the same environmental justice concerns as fossil fuels, except for their greenhouse benefits. Environmental justice concerns thus reinforce the need to electrify transport as soon as possible (including hydrogen fuel cell heavy trucks). These environmental justice concerns are discussed extensively in Chapters 3 and 9. On the other hand, biofuels offer employment and revenue in rural communities, many of which struggle economically. However, BECCS hydrogen or electricity could provide much the same benefits.
The IIJA includes $500 million for biofuels infrastructure (Section 11401), while the IRA contains about $6 billion of biofuels incentives (BPC 2022), a $6.5 billion total investment mostly devoted to biofuels production. The IRA extends the current $1/gallon tax credit for biodiesel until 2024, and then replaces it with a credit of $0.2/gallon for biofuels with 50 percent greenhouse efficiency, like current biodiesel (where greenhouse efficiency equals the percent of the GHG emissions of an energy-equivalent amount of fossil fuel). This credit increases linearly with the greenhouse efficiency of the fuel, until
it reaches $1/gallon for fuels with zero emissions. The IRA also includes a tax credit for aviation biofuels that starts at $1.25/gallon for 50 percent greenhouse efficiency and increases to $1.75 gallon for fuels with zero greenhouse emissions (BPC 2022).
BECCS is also implicitly subsidized by the Carbon Capture and Sequestration Tax Credit (45Q) incentives for CCUS ($85/tCO2 for CCS and $60/tCO2 for CCU), and biohydrogen by the New Clean Hydrogen Production Tax Credit (45V) incentives (ranging from $0.6/kgH2 to $3/kgH2 depending on the greenhouse intensity of the fuel). These incentives are large enough when combined with other existing incentives, such as California’s low carbon fuel subsidy, to make some biofuels highly cost competitive (Cheng et al. 2023). However, other analyses of the bills see relatively little expansion of biofuels production during the 2020s because of the time and capital required to scale up production, the uncertainty of future demand given the progressive electrification of transport, and uncertainty about federal and state incentives (Jenkins et al. 2022; Mahajan et al. 2022).
The IRA, IIJA, and CHIPS do not include funding for BECCS demonstration projects, and DOE has not yet announced a specific plan to develop BECCS. This is a critical shortcoming, given the system complexity of BECCS and the potential need for relatively cheap and efficient BECCS after 2030. Also, Recommendation 9-6 in Chapter 9 calls for increased research on advanced liquid fuels in general, given the long-term needs of aviation and other models of transport that prove difficult to electrify economically.
Finding 8-3: The $6.5 billion of biofuels-related funding in the IRA and IIJA is sufficient for biofuels production in the 2020s and for research and development (R&D) so that advanced land transport and aviation biofuels are ready after 2030. However, there is not currently a sufficient comprehensive plan to develop BECCS.
Finding 8-4: The need for carbon sinks and net-zero chemical fuels during 2030–2050 would likely cause a rush to biomass production that would decrease agricultural and forest land. This could impact food prices around the world, with implications for political stability, and could cause deforestation with adverse effects on biodiversity and increased carbon emissions.
Recommendation 8-7: Release a Comprehensive Research, Development, Demonstration, and Deployment (RDD&D) Program for Biomass Energy with Carbon Capture and Storage (BECCS). The Department of Energy should complete and release a comprehensive RDD&D program for BECCS based on the recommendations in the 2019 National Academies’ report Negative Emissions Technologies and Reliable Sequestration: A Research Agenda. This should include both biomass to electricity and biomass to fuels.
EMISSIONS AND LAND USE RELATED TO FOOD AND DIETS
Reducing Food Waste
Food waste is also a major environmental and economic challenge, with approximately one-third of U.S. food never eaten, representing a significant waste of resources. The GHG emissions embodied in this food waste amount annually to 170 Mt CO2e (excluding landfill emissions, where food is the single most common material and decomposes into methane) (Read et al. 2020). The majority of these emissions occur during the process of growing and harvesting food, so source reduction (preventing food overproduction and subsequent diversion to another use) is a more effective method to reduce emissions than methods that recycle food into another use such as animal feed, compost, or anaerobic digestion (EPA 2021). Because roughly half of food waste in the United States occurs at the consumption stage (in households and restaurants), and because embodied emissions accumulate as food progresses through the supply chain, reducing food waste at the consumption stage can result in the greatest emissions reductions. Pathways to reduce food waste at the consumer level include (1) changing the U.S. food environment to discourage waste, (2) strengthening consumers’ ability and motivation to reduce food waste, and (3) leveraging and applying research and technology to support consumers in food waste reduction (NASEM 2020). Additionally, reducing waste of animal products such as dairy and beef—whose production emits a relatively greater amount of GHGs per unit of food—can result in greater reductions of emissions than reducing a similar amount of other foods (EPA 2021). EPA has identified addressing food waste as a priority area for reducing GHG emissions, with a national goal to halve food waste by 2030, which could reduce food sector emissions by roughly 10 percent. While the nation has not yet made significant progress toward this goal, if it were achieved, emissions from the food system could be reduced by 92 MtCO2e/y (EPA 2021; Read et al. 2020), so efforts to make progress on reducing food waste could be a part of national net-zero emissions strategy.
Finding 8-5: Reducing food waste could be an important way to reduce food sector GHG emissions more broadly. If food waste were halved, up to 10 percent of emissions from the food system could be mitigated.
Land Use Requirements and Emissions Related to Animal Agriculture
Multiple indicators need to be considered in assessing dietary sustainability (e.g., overall nutritional quality, GHG emissions, water and land use, economic cost, health),
and most of these indicators are dependent on the type of food produced and the efficiency of production (Chen et al. 2019). It follows that the composition of the human diet is inextricably linked to all of these indicators, and significantly, environmental impact. Even if fossil fuel emissions were to be eliminated entirely, current trends in food systems might prevent the achievement of the 1.5 degree Paris Agreement target (Clark et al. 2020). Animal agriculture specifically accounts for 5 percent of global carbon dioxide emissions, 37–44 percent of global methane emissions, 44 percent of nitrous oxide emissions, and 75–80 percent of total agricultural emissions (FAO et al. 2021). Furthermore, approximately 50 percent of global habitable land is currently used for agriculture; however, that percentage varies by country depending on dietary patterns, which are strongly correlated to a country’s gross domestic product (Alexander et al. 2016). Of the finite amount of land available for agriculture, 77 percent (~4 Bha) is in use today for animal agriculture, either for animal feed production or grazing (Figure 8-8). This percentage will only grow as the global population continues to grow, so reducing per capita consumption of livestock, meat, and dairy both globally and in the United States will be critical to stay within both the arable land and emissions budgets for the agriculture sector (Willet et al. 2019).
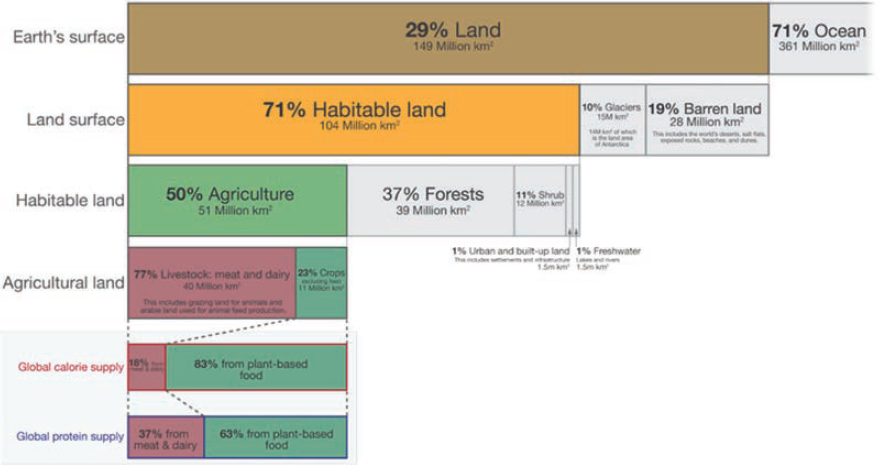
In the United States, approximately 75–80 percent of protein consumption comes from animal sources, making up only 18 percent of total calorie consumption. Furthermore, within diets in the United States, meat and dairy constitute 75 percent of all emissions from food production and consumption (Heller et al. 2018). In addition to the emission profile of animal products, conventional meat supply chains pose other environmental and dietary health impacts, discussed in Chapter 3.
Alternative ways to produce protein could reduce environmental and health impacts in addition to addressing the disproportionate level of land and resource use required by animal agriculture. Eighty-five percent of harvested soybean and grain is fed to animals each year; if soybeans and grains were directly fed to humans, farm acreage could be dramatically reduced, and land could be freed up for biofuels, sequestration, and other purposes (Cassidy et al. 2013). Hayek et al. (2021) mapped the magnitude of the carbon opportunity cost of resource-intensive diets and found that a global shift to more plant-based diets could lead to the sequestration of 332–547 GtCO2 by 2050, providing evidence that shifts to plant-based diets could lead to greater mitigation options. Eliminating the cycling of calories through animals represents a huge opportunity to reinvent the agricultural supply chain in the United States, which is based disproportionally on supporting livestock. Directly converting protein from crops to human food redefines the value proposition of the agricultural supply chain.
Finding 8-6: If plant-based substitutes were to substantially replace meat and dairy, this would fundamentally change net-zero strategies, as a significant portion of arable land could then be used for other purposes (e.g., carbon sinks, biofuels).
Meat and Dairy Alternatives and Market Potential
Meat Substitutes
Today, the plant-based meat industry in the United States totals about $1.4 billion (Grand View Research 2023). With many meat substitutes already on the market and looking to grow, other innovative technologies are paving the way to producing “real” meat without the animal. There are three main alternative protein technologies: (1) protein isolates of traditional foods (e.g., legumes, grains); (2) precision fermentation proteins (e.g., fungal and yeast); and (3) cell-based and cultured proteins (“tissue engineering”). Hybrid products that leverage several of these processes are also being explored. The products that result from these technologies require regulatory evaluation by the Food and Drug Administration and USDA to establish safety, and investments in R&D as well as supply chain and manufacturing.
Protein isolates and fermentation products have a long history in the food supply chain, and many meat substitutes made from these materials have been on the market for decades. Recent years have seen an uptick in interest for meat-mimicking foods made by these processes, with companies like Beyond® and Impossible® creating commercial products that are already close to price parity with conventional meat.10 This, however, has not significantly altered the market share of conventional meat. While the market for plant-based meat substitutes is projected to grow by 11 percent by 2029 (Fortune Business Insights 2022), the much larger conventional meat market ($3 trillion, globally) is also expected to grow by 5.7 percent. The effects of a growing meat-substitute market on cattle production will likely be small unless meat substitutes can capture much more of the market than these projections indicate (Lusk et al. 2022).11
Cell-based and cultured meat products are still a developing but potentially disruptive technology with cost curves not yet amenable to widespread commercialization. However, there are currently more than 70 companies working on this technology (Bandoim 2022), and prices have fallen precipitously over the past decade, similar to cost and performance advances in computing technologies governed by Moore’s law (Shigeta 2020). Achieving cost parity with conventional meat production could be realized by 2030 with sufficient R&D and investments in supply chain (Vergeer et al. 2021).
Should cultivated meat products reach price parity, their impact on the market share of conventional meat will still depend on a variety of sociological and cultural factors. Consumer ideology and culture will greatly affect interest or aversion to new types of food, even foods that replicate traditional products down to the DNA. Global protein needs will increase substantially by 2050, and policies and incentives that take consumer ideologies, anxieties, and taste preferences into account will be necessary to
___________________
10 A recent scenario-driven assessment of the plant-based meat industry’s future suggests that manufacturing capacity (not raw ingredients) is expected to be rate-limiting, and the industry will need to invest $27 billion in global capital expenditure to meet projected global production needs and a minimum of $17 billion in annual operating costs (Troya et al. 2022).
11 An economic model estimating how a reduction in price or increase in demand for plant-based meat in the United States affects cattle production found that increases in U.S. demand alter trade patterns, leading to a reduction of beef imports and an increase in beef exports, which reduces emissions and land use given the relative efficiency of U.S. beef production. The study found that for every 10 percent reduction in price, the global reduction in emissions is equivalent to 0.34 percent of U.S. emissions from beef production and 1.14 percent when including reduced land use change emissions, such that even substantial reductions in prices are unlikely to have substantive impacts on the U.S. cattle population and emissions (Lusk 2022). This suggests the need to also pursue alternative mitigation strategies, such as innovations to reduce methane emissions per head.
facilitate a transition to more sustainable diets (Meybeck and Gitz 2017; Yin et al. 2020). For example, although unprocessed plant-based foods are often cheaper than animal foods, most consumers today prefer to keep meat in their diet despite higher costs, higher environmental impact, and potential higher health impacts.
Dairy Substitutes
While the GHG and resource footprint is highest for beef cattle than any other animal product, dairy is a large emissions contributor that cannot be overlooked. The dairy and beef industries are intertwined, but the mitigation potential of dairy alone is likely only about one-third that of beef. However, alternative dairy products are currently viewed differently (and more favorably) by consumers than meat substitutes, making dairy substitutes an important mitigation opportunity (NASEM 2023). Plant-based milks are currently the largest plant-based category of foods in North America, and currently account for 10 percent of the market (Hale 2021).
Finding 8-7: Owing to a variety of cultural and sociological factors, demand growth for meat substitutes may be too slow to have major impact on land use and GHG emissions before 2050, especially considering what is known about current consumer behavior. There is potentially more opportunity but less mitigation benefit with dairy substitutes.
Despite the current animal protein–oriented food system’s high GHG emissions, potential adverse health impacts, and high demand for arable land, research shows that a fundamental change in the food animal production system will be difficult, requiring technological innovation, policies that make sustainable food more accessible and affordable, and buy-in from consumers. There is no investment in alternative protein technologies in the IRA. There is an opportunity in the Farm Bill to invest in R&D, and in business and consumer incentives for meat and dairy substitutes, as well as to increase incentives for whole foods such as fruits, vegetables, and legumes.
Recommendation 8-8: Convene an Expert Group to Recommend Policies That Could Encourage Sustainable Diets. The Secretary of Agriculture should quickly convene an expert group to recommend policies that could facilitate societal acceptance and adoption of sustainable diets with lower consumption of animal products and increased consumption of plant-based foods.
All options should be considered, including some potentially politically difficult options—for example, the following:
- Including food in a comprehensive tax on greenhouse gas (GHG) emissions (while addressing the impacts on consumers with lower incomes).
- Creating federal incentives for plant-based meat substitutes and cell-cultivated products that reflect their life-cycle GHG and land use benefits relative to conventional meat.
- Including sustainability in U.S. Department of Agriculture dietary guidelines, and supporting data collection and availability on the life-cycle environmental impacts of emerging food products.
- Including sustainability in federal food procurement requirements.
- Phasing-out programs that incentivize the production or promotion of high-emission conventional meat products, while supporting just transitions for farms and workers.
Table 8-3 summarizes all of the recommendations in this chapter regarding land use in support of decarbonization efforts.
SUMMARY OF RECOMMENDATIONS ON LAND USE
TABLE 8-3 Summary of Recommendations on Land Use
Short-Form Recommendation | Actor(s) Responsible for Implementing Recommendation | Sector(s) Addressed by Recommendation | Objective(s) Addressed by Recommendation | Overarching Categories Addressed by Recommendation |
---|---|---|---|---|
8-1: Convene an Expert Group to Recommend Ways to Measure Additional Forest Sinks | Secretary of Agriculture |
|
|
Rigorous and Transparent Analysis and Reporting for Adaptive Management |
8-2: Prioritize Ecosystem-Level Carbon Storage | Secretary of Agriculture |
|
|
A Broadened Policy Portfolio |
8-3: Establish a Permanent, National-Scale, High-Quality Soil Monitoring Network | U.S. Department of Agriculture (USDA) |
|
|
Rigorous and Transparent Analysis and Reporting for Adaptive Management |
8-4: Build Out Long-Term Agricultural Field Experiments | USDA |
|
|
Rigorous and Transparent Analysis and Reporting for Adaptive Management Research, Development, and Demonstration Needs |
8-5: Fund Research to Quantify Indicators That Influence Adoption of Regenerative Agriculture Practices | USDA |
|
|
Research, Development, and Demonstration Needs |
Short-Form Recommendation | Actor(s) Responsible for Implementing Recommendation | Sector(s) Addressed by Recommendation | Objective(s) Addressed by Recommendation | Overarching Categories Addressed by Recommendation |
---|---|---|---|---|
8-6: Incentivize the Abatement of CH4 and N2O Emissions and Improve Soil Carbon Sequestration | USDA |
|
|
A Broadened Policy Portfolio |
8-7: Release a Comprehensive Research, Development, Demonstration, and Deployment Program for Biomass Energy with Carbon Capture and Storage | Department of Energy |
|
|
Research, Development, and Demonstration Needs |
8-8: Convene an Expert Group to Recommend Policies That Could Encourage Sustainable Diets | Secretary of Agriculture |
|
|
A Broadened Policy Portfolio |
REFERENCES
Achinas, S., J. Horjus, V. Achinas, and G.J.W. Euverink. 2019. “A PESTLE Analysis of Biofuels Energy Industry in Europe.” Sustainability 11(21):5981. https://doi.org/10.3390/su11215981.
Ager, A.A., M.A. Finney, A. McMahan, and J. Cathcart. 2010. “Measuring the Effect of Fuel Treatments on Forest Carbon Using Landscape Risk Analysis.” Natural Hazards and Earth System Sciences 10(12):2515–2526. https://doi.org/10.5194/nhess-10-2515-2010.
Alexander, P., C. Brown, A. Arneth, J. Finnigan, and M.D.A. Rounsevell. 2016. “Human Appropriation of Land for Food: The Role of Diet.” Global Environmental Change. http://www.sciencedirect.com/science/article/pii/S0959378016302370.
Bagnall, D.K., J.F. Shanahan, A. Flanders, C.L.S. Morgan, and C.W. Honeycutt. 2021. “Soil Health Considerations for Global Food Security.” Agronomy Journal 113(6):4581–4589. https://doi.org/10.1002/agj2.20783.
Bandoim, L. 2022. “Making Meat Affordable: Progress Since the $330,000 Lab-Grown Burger.” Forbes, March 8. https://www.forbes.com/sites/lanabandoim/2022/03/08/making-meat-affordable-progress-since-the-330000-lab-grown-burger.
Beerling, D.J., E.P. Kantzas, M.R. Lomas, P. Wade, R.M. Eufrasio, P. Renforth, B. Sarkar, et al. 2020. “Potential for Large-Scale CO2 Removal via Enhanced Rock Weathering with Croplands.” Nature 583(7815):242–248. https://doi.org/10.1038/s41586-020-2448-9.
Bellemare, M.F. 2015. “Rising Food Prices, Food Price Volatility, and Social Unrest.” American Journal of Agricultural Economics 97(1):1–21. https://doi.org/10.1093/ajae/aau038.
Birdsey, R.A., D.A. DellaSala, W.S. Walker, S.R. Gorelik, G. Rose, and C.E. Ramírez. 2023. “Assessing Carbon Stocks and Accumulation Potential of Mature Forests and Larger Trees in US Federal Lands.” Frontiers in Forests and Global Change 5(277).
BPC (Bipartisan Policy Center). 2022. “Inflation Reduction Act Summary.” https://bipartisanpolicy.org/download/?file=/wp-content/uploads/2022/08/Energy-IRA-Brief_R04-9.26.22.pdf.
California Natural Resources Agency. n.d. “Expanding Nature-Based Solutions.” https://resources.ca.gov/Initiatives/Expanding-Nature-Based-Solutions. Accessed February 17, 2023.
Calvert, K.E. 2018. “Measuring and Modelling the Land-Use Intensity and Land Requirements of Utility-Scale Photovoltaic Systems in the Canadian Province of Ontario: Solar Energy Land Use.” Canadian Geographer/Le Géographe Canadien 62(2):188–199. https://doi.org/10.1111/cag.12444.
Calvin, K., M. Wise, P. Kyle, P. Patel, L. Clarke, and J. Edmonds. 2014. “Trade-Offs of Different Land and Bioenergy Policies on the Path to Achieving Climate Targets.” Climatic Change 123(3):691–704. https://doi.org/10.1007/s10584-013-0897-y.
Campbell, J.L., M.E. Harmon, and S.R. Mitchell. 2012. “Can Fuel-Reduction Treatments Really Increase Forest Carbon Storage in the Western US by Reducing Future Fire Emissions?” Frontiers in Ecology and the Environment 10(2):83–90. https://doi.org/10.1890/110057.
Capellán-Pérez, I., C. De Castro, and I. Arto. 2017. “Assessing Vulnerabilities and Limits in the Transition to Renewable Energies: Land Requirements Under 100% Solar Energy Scenarios.” Renewable and Sustainable Energy Reviews 77:760–782. https://doi.org/10.1016/j.rser.2017.03.137.
Cassidy, E.S., P.C. West, J.S. Gerber, and J.A. Foley. 2013. “Redefining Agricultural Yields: From Tonnes to People Nourished per Hectare.” Environmental Research Letters 8(3):034015. https://doi.org/10.1088/1748-9326/8/3/034015.
Chambers, A., R. Lal, and K. Paustian. 2016. “Soil Carbon Sequestration Potential of US Croplands and Grasslands: Implementing the 4 per Thousand Initiative.” Journal of Soil and Water Conservation 71(3):68A–74A. https://doi.org/10.2489/jswc.71.3.68A.
Chen, C., A. Chaudhary, and A. Mathys. 2019. “Dietary Change Scenarios and Implications for Environmental, Nutrition, Human Health and Economic Dimensions of Food Sustainability.” Nutrients 11(4):856. https://doi.org/10.3390/nu11040856.
Cheng, F., H. Luo, J.D. Jenkins, and E.D. Larson. 2023. “The Value of Low- and Negative-Carbon Fuels in the Transition to Net-Zero Emission Economies: Lifecycle Greenhouse Gas Emissions and Cost Assessments Across Multiple Fuel Types.” Applied Energy 331:120388. https://doi.org/10.1016/j.apenergy.2022.120388.
Chiono, L.A., D.L. Fry, B.M. Collins, A.H. Chatfield, and S.L. Stephens. 2017. “Landscape-Scale Fuel Treatment and Wildfire Impacts on Carbon Stocks and Fire Hazard in California Spotted Owl Habitat.” Ecosphere 8(1):e01648. https://doi.org/10.1002/ecs2.1648.
Clark, M.A., N.G.G. Domingo, K. Colgan, S.K. Thakrar, D. Tilman, J. Lynch, I.L. Azevedo, and J.D. Hill. 2020. “Global Food System Emissions Could Preclude Achieving the 1.5° and 2°C Climate Change Targets.” Science 370(6517):705–708. https://doi.org/10.1126/science.aba7357.
Coffield, S.R., C.D. Vo, J.A. Wang, G. Badgley, M.L. Goulden, D. Cullenward, W.R.L. Anderegg, and J.T. Randerson. 2022. “Using Remote Sensing to Quantify the Additional Climate Benefits of California Forest Carbon Offset Projects.” Global Change Biology 28(22):6789–6806. https://doi.org/10.1111/gcb.16380.
Cook-Patton, S.C., R.C. Drever, K. Hamrick, H. Hardman, T. Kroeger, S. Yeo, P.W. Ellis, et al. 2021a. “Protect, Manage and Then Restore Lands for Climate Mitigation.” Nature Climate Change 11(12):1027–1034. https://doi.org/10.1038/s41558-021-01198-0.
Cook-Patton, S.C., D. Shoch, and P.W. Ellis. 2021b. “Dynamic Global Monitoring Needed to Use Restoration of Forest Cover as a Climate Solution.” Nature Climate Change 11(5):366–368. https://doi.org/10.1038/s41558-021-01022-9.
Coyle, W.T. 2007. “The Future of Biofuels: A Global Perspective.” U.S. Department of Agriculture Economic Research Service. November 1. https://www.ers.usda.gov/amber-waves/2007/november/the-future-of-biofuels-a-global-perspective.
DOE (Department of Energy). 2022a. “Clean Cities Alternative Fuel Price Report.” DOE Office of Energy Efficiency and Renewable Energy. https://afdc.energy.gov/files/u/publication/alternative_fuel_price_report_july_2022.pdf.
DOE. 2022b. “U.S. Corn Production and Portion Used for Fuel Ethanol.” DOE Office of Energy Efficiency and Renewable Energy. https://afdc.energy.gov/data/10339.
DOE. n.d.(a). “Biodiesel Production and Blending Tax Credit.” Alternative Fuels Data Center. https://afdc.energy.gov/laws/5831. Accessed February 17, 2023.
DOE. n.d.(b). “Biodiesel Vehicle Emissions.” Alternative Fuels Data Center. https://afdc.energy.gov/vehicles/diesels_emissions.html. Accessed February 17, 2023.
DOE. n.d.(c). “Biofuels and Greenhouse Gas Emissions: Myths Versus Facts.” https://www.energy.gov/sites/prod/files/edg/media/BiofuelsMythVFact.pdf. Accessed February 17, 2023.
DOI (Department of the Interior). 2016. “Fundamental Considerations Associated with Placing Solar Generation Structures at Central Arizona Project Canal.”
Domingo, N.G.G., S. Balasubramanian, S.K. Thakrar, M.A. Clark, P.J. Adams, J.D. Marshall, N.Z. Muller, et al. 2021. “Air Quality–Related Health Damages of Food.” Proceedings of the National Academy of Sciences 118(20):e2013637118. https://doi.org/10.1073/pnas.2013637118.
DOS and EOP (Department of State and Executive Office of the President). 2021. The Long-Term Strategy of the United States: Pathways to Net-Zero Greenhouse Gas Emissions by 2050. https://www.whitehouse.gov/wp-content/uploads/2021/10/US-Long-Term-Strategy.pdf.
Eagle, A.J., A.L. Hughes, N.A. Randazzo, C.L. Schneider, C.H. Melikov, E. Puritz, K. Jaglo, and B. Hurley. 2022. Ambitious Climate Mitigation Pathways for U.S. Agriculture and Forestry: Vision for 2030. New York: Environmental Defense Fund and Washington, DC: ICF International. https://www.edf.org/sites/default/files/documents/climate-mitigation-pathways-us-agriculture-forestry.pdf.
Ecosystem Marketplace. 2021. Markets in Motion: State of Voluntary Carbon Markets 2021, Installment 1. Washington, DC: Forest Trends Association. https://www.ecosystemmarketplace.com/publications/state-of-the-voluntary-carbon-markets-2021.
EIA (U.S. Energy Information Administration). 2018. “EPA Finalizes Renewable Fuel Standard for 2019, Reflecting Cellulosic Biofuel Shortfalls.” https://www.eia.gov/todayinenergy/detail.php?id=37712.
EIA. 2019. “EIA Projects Energy Consumption in Air Transportation to Increase Through 2050.” Today In Energy, November 6. https://www.eia.gov/todayinenergy/detail.php?id=41913.
EPA (Environmental Protection Agency). 2019. “Global Non-CO2 Greenhouse Gas Emission Projections and Mitigation 2015–2050.” EPA-430-R-19-010. https://www.epa.gov/global-mitigation-non-co2-greenhouse-gases/global-non-co2-greenhouse-gas-emission-projections.
EPA. 2021. “From Farm to Kitchen: The Environmental Impacts of US Food Waste.” EPA 600-R21 171. https://www.epa.gov/system/files/documents/2021-11/from-farm-to-kitchen-the-environmental-impacts-of-u.s.-food-waste_508-tagged.pdf.
EPA. 2022. “Inventory of U.S. Greenhouse Gas Emissions and Sinks: 1990–2020.” EPA-430-R-22-003. https://www.epa.gov/system/files/documents/2022-04/us-ghg-inventory-2022-main-text.pdf.
EPA. n.d.(a). “Greenhouse Gas Inventory Data Explorer.” https://cfpub.epa.gov/ghgdata/inventoryexplorer/#iagriculture/entiresector/allgas/gas/all. Accessed February 15, 2023.
EPA. n.d.(b). “Overview of Greenhouse Gases.” https://www.epa.gov/ghgemissions/overview-greenhouse-gases. Accessed February 15, 2023.
FAO (Food and Agriculture Organization), IFAD (International Fund for Agricultural Development), UNICEF (United Nations Children’s Fund), WFP (World Food Programme), and WHO (World Health Organization). 2021. The State of Food Security and Nutrition in the World 2021. Rome, Italy: Food and Agriculture Organization. https://doi.org/10.4060/cb4474en.
Fargione, J., J. Hill, D. Tilman, S. Polasky, and P. Hawthorne. 2008. “Land Clearing and the Biofuel Carbon Debt.” Science 319(5867):1235–1238. https://doi.org/10.1126/science.1152747.
Fargione, J., S. Bassett, T. Boucher, S.D. Bridgham, R.T. Conant, S.C. Cook-Patton, P.W. Ellis, et al. 2018. “Natural Climate Solutions for the United States.” Science Advances 4(11):eaat1869. https://doi.org/10.1126/sciadv.aat1869.
Fargione, J., D.L. Haase, O.T. Burney, O.A. Kildisheva, G. Edge, S.C. Cook-Patton, T. Chapman, et al. 2021. “Challenges to the Reforestation Pipeline in the United States.” Frontiers in Forests and Global Change 4(February):629198. https://doi.org/10.3389/ffgc.2021.629198.
FBLE (Farm Bill Law Enterprise). 2022. “Equity in Agricultural Production and Governance.” https://www.farmbilllaw.org/wp-content/uploads/2022/10/Equity-Report.pdf.
Fortune Business Insights. 2022. “Meat Substitutes Market Size, Share and COVID-19 Impact Analysis, by Source (Soy-Based Ingredients, Wheat-Based Ingredients, Other Grain-Based Ingredients, and Textured Vegetable Proteins), by Distribution Channel (Mass Merchandisers, Specialty Stores, Online Retail, Other Retail Channels, and Food Service), and Regional Forecast, 2022–2029.” FBI100239. https://www.fortunebusinessinsights.com/industry-reports/meat-substitutes-market-100239.
Foster, D.E., J.J. Battles, B.M. Collins, R.A. York, and S.L. Stephens. 2020. “Potential Wildfire and Carbon Stability in Frequent-Fire Forests in the Sierra Nevada: Trade-Offs from a Long-Term Study.” Ecosphere 11(8). https://doi.org/10.1002/ecs2.3198.
Fraunhofer Institute for Solar Energy Systems ISE. 2020. Agrivoltaics: Opportunities for Agriculture and the Energy Transition: A Guideline for Germany. Freiburg, Germany.
Glover, J.D., J.P. Reganold, L.W. Bell, J. Borevitz, E.C. Brummer, E.S. Buckler, C.M. Cox, et al. 2010. “Increased Food and Ecosystem Security via Perennial Grains.” Science 328(5986):1638–1639. https://doi.org/10.1126/science.1188761.
Grand View Research. 2023. “Plant-Based Meat Market.” https://www.grandviewresearch.com/industry-analysis/plant-based-meat-market.
Hale, M. 2021. “Exploring the Growth of Plant-Based Milk.” Food Manufacturing, September 23. https://www.foodmanufacturing.com/consumer-trends/article/21723117/exploring-the-growth-of-plantbased-milk.
Hayek, M.N., H. Harwatt, W.J. Ripple, and N.D. Mueller. 2021. “The Carbon Opportunity Cost of Animal-Sourced Food Production on Land.” Nature Sustainability 4(1):21–24. https://doi.org/10.1038/s41893-020-00603-4.
Heller, M.C, A. Willits-Smith, R. Meyer, G.A. Keoleian, and D. Rose. 2018. “Greenhouse Gas Emissions and Energy Use Associated with Production of Individual Self-Selected US Diets.” Environmental Research Letters 13(4):044004. https://doi.org/10.1088/1748-9326/aab0ac.
Hoover, C.M., and J.E. Smith. 2021. “Current Aboveground Live Tree Carbon Stocks and Annual Net Change in Forests of Conterminous United States.” Carbon Balance and Management 16(1):17. https://doi.org/10.1186/s13021-021-00179-2.
Horst, M., and A. Marion. 2019. “Racial, Ethnic and Gender Inequities in Farmland Ownership and Farming in the U.S.” Agriculture and Human Values 36(1):1–16. https://doi.org/10.1007/s10460-018-9883-3.
Hudiburg, T.W., B.E. Law, C. Wirth, and S. Luyssaert. 2011. “Regional Carbon Dioxide Implications of Forest Bioenergy Production. Nature Climate Change 1:419–423. https://doi.org/10.1038/nclimate1264.
Hudiburg, T.W., B.E. Law, W.R. Moomaw, M.E. Harmon, and J.E. Stenzel. 2019. “Meeting GHG Reduction Targets Requires Accounting for All Forest Sector Emissions. Environmental Research Letters 14:095005. https://doi.org/10.1088/1748-9326/ab28bb.
Hurteau, M.D., and M.L. Brooks. 2011. “Short- and Long-Term Effects of Fire on Carbon in US Dry Temperate Forest Systems.” BioScience 61(2):139–146. https://doi.org/10.1525/bio.2011.61.2.9.
Hurtt, G.C., S.W. Pacala, P.R. Moorcroft, J. Caspersen, E. Shevliakova, R.A. Houghton, and B. Moore. 2002. “Projecting the Future of the U.S. Carbon Sink.” Proceedings of the National Academy of Sciences 99(3):1389–1394. https://doi.org/10.1073/pnas.012249999.
IPCC (Intergovernmental Panel on Climate Change). 2022. Global Warming of 1.5°C: IPCC Special Report on Impacts of Global Warming of 1.5°C Above Pre-Industrial Levels in Context of Strengthening Response to Climate Change, Sustainable Development, and Efforts to Eradicate Poverty, 1st ed., V. Masson-Delmotte, P. Zhai, H.-O. Pörtner, et al., eds. Cambridge, UK, and New York: Cambridge University Press. https://doi.org/10.1017/9781009157940.
Jacobson, M.Z., and M.A. Delucchi. 2011. “Providing All Global Energy with Wind, Water, and Solar Power, Part I: Technologies, Energy Resources, Quantities and Areas of Infrastructure, and Materials.” Energy Policy 39(3):1154–1169. https://doi.org/10.1016/j.enpol.2010.11.040.
Jacobson, M.Z., M.A. Delucchi, M.A. Cameron, and B.A. Frew. 2015. “Low-Cost Solution to the Grid Reliability Problem with 100% Penetration of Intermittent Wind, Water, and Solar for All Purposes.” Proceedings of the National Academy of Sciences 112(49):15060–15065. https://doi.org/10.1073/pnas.1510028112.
Jenkins, J.D., E.N. Mayfield, E.D. Larson, S.W. Pacala, and C. Greig. 2021. “Mission Net-Zero America: The Nation-Building Path to a Prosperous, Net-Zero Emissions Economy.” Joule 5(11):2755–2761. https://doi.org/10.1016/j.joule.2021.10.016.
Jenkins, J.D., E.N. Mayfield, J. Farbes, R. Jones, N. Patankar1, Q. Xu, and G. Schivley. 2022. “Preliminary Report: The Climate and Energy Impacts of the Inflation Reduction Act of 2022.” Princeton, NJ: REPEAT Project, p. 17. https://repeatproject.org/docs/REPEAT_IRA_Prelminary_Report_2022-08-04.pdf.
Jennings, R., A.D. Henderson, A. Phelps, K.M. Janda, and A.E. Van Den Berg. 2023. “Five U.S. Dietary Patterns and Their Relationship to Land Use, Water Use, and Greenhouse Gas Emissions: Implications for Future Food Security.” Nutrients 15(1):215. https://doi.org/10.3390/nu15010215.
Kreidenweis, U., F. Humpenöder, M. Stevanović, B.L. Bodirsky, E. Kriegler, H. Lotze-Campen, and A. Popp. 2016. “Afforestation to Mitigate Climate Change: Impacts on Food Prices Under Consideration of Albedo Effects.” Environmental Research Letters 11(8):085001. https://doi.org/10.1088/1748-9326/11/8/085001.
Larsen, J., B. King, E. Wimberger, H. Pitt, H. Kolus, A. Rivera, N. Dasari, C. Jahns, K. Larsen, and W. Herndon. 2021. “Pathways to Paris: A Policy Assessment of the 2030 US Climate Target.” Rhodium Group. https://rhg.com/research/us-climate-policy-2030.
Larsen, J., B. King, H. Kolus, N. Dasari, G. Hiltbrand, and W. Herndon. 2022. “A Turning Point for US Climate Progress: Assessing the Climate and Clean Energy Provisions in the Inflation Reduction Act.” Rhodium Group. https://rhg.com/research/climate-clean-energy-inflation-reduction-act.
Larson, E., C. Greig, J. Jenkins, E. Mayfield, A. Pascale, C. Zhang, J. Drossman, et al. 2020. Net-Zero America: Potential Pathways, Infrastructure, and Impacts, Interim Report. Princeton, NJ: Princeton University. https://netzeroamerica.princeton.edu/img/Princeton_NZA_Interim_Report_15_Dec_2020_FINAL.pdf.
Larson, E., C. Greig, J. Jenkins, E. Mayfield, A. Pascale, C. Zhang, J. Drossman, et al. 2021. Net-Zero America: Potential Pathways, Infrastructure, and Impacts, Final Report.” Princeton, NJ: Princeton University. https://netzeroamerica.princeton.edu/the-report.
Law, B.E., T.W. Hudiburg, L.T. Berner, J.J. Kent, P.C. Buotte, and M.E. Harmon. 2018. “Land Use Strategies to Mitigate Climate Change in Carbon Dense Temperate Forests.” Proceedings of the National Academy of Sciences 115(14):3663–3668.
Law, B.E., W.R. Moomaw, R.W. Hudiburg, W.H. Schlesinger, J.D. Sterman, and G.M. Woodwell. 2022. “Creating Strategic Reserves to Protect Forest Carbon and Reduce Biodiversity Losses in the United States.” Land 11:721.
Lempert, R., B.L. Preston, J. Edmonds, L. Clarke, T. Wild, M. Binsted, E. Diringer, and B. Townsend. 2019. “Pathways to 2050: Scenarios for Decarbonizing the U.S. Economy.” Center for Climate and Energy Solutions. https://www.c2es.org/document/pathways-to-2050-scenarios-for-decarbonizing-the-u-s-economy.
Li, Y., C.K. Miskin, and R. Agrawal. 2018. “Land Availability, Utilization, and Intensification for a Solar Powered Economy.” Computer Aided Chemical Engineering 44:1915–1920. https://doi.org/10.1016/B978-0-444-64241-7.50314-1.
Lusk, J.L., D. Blaustein-Rejto, S. Shah, and G.T. Tonsor. 2022. “Impact of Plant-Based Meat Alternatives on Cattle Inventories and Greenhouse Gas Emissions.” Environmental Research Letters, January. https://doi.org/10.1088/1748-9326/ac4fda.
Mahajan, M., O. Ashmoore, J. Rissman, R. Orvis, and A. Gopal. 2022. “Updated Inflation Reduction Act Modeling Using the Energy Policy Simulator.” Energy Innovation. https://energyinnovation.org/wp-content/uploads/2022/08/Updated-Inflation-Reduction-Act-Modeling-Using-the-Energy-Policy-Simulator.pdf.
Meybeck, A., and V. Gitz. 2017. “Sustainable Diets Within Sustainable Food Systems.” Proceedings of the Nutrition Society 76(1):1–11. https://doi.org/10.1017/S0029665116000653.
Mitchell, S.R., M.E. Harmon, and K.E.B. O’Connell. 2012. “Carbon Debt and Carbon Sequestration Parity in Forest Bioenergy Production.” GCB Bioenergy.
NASEM (National Academies of Sciences, Engineering, and Medicine). 2019. Negative Emissions Technologies and Reliable Sequestration: A Research Agenda. Washington, DC: The National Academies Press. https://doi.org/10.17226/25259.
NASEM. 2020. A National Strategy to Reduce Food Waste at the Consumer Level. Washington, DC: The National Academies Press. https://doi.org/10.17226/25876.
NASEM. 2021. Accelerating Decarbonization of the U.S. Energy System. Washington, DC: The National Academies Press. https://doi.org/10.17226/25932.
NASEM. 2023. Alternative Protein Sources: Balancing Food Innovation, Sustainability, Nutrition, and Health: Proceedings of a Workshop. Washington, DC: The National Academies Press. https://doi.org/10.17226/26923.
Network Rail. 2023. “Thameslink Programme.” https://www.networkrail.co.uk/running-the-railway/railway-upgrade-plan/key-projects/thameslink-programme.
North, M., M. Hurteau, and J. Innes. 2009. “Fire Suppression and Fuels Treatment Effects on Mixed-Conifer Carbon Stocks and Emissions.” Ecological Applications 19(6):1385–1396. https://doi.org/10.1890/08-1173.1.
Novick, K.A., S. Metzger, W.R.L. Anderegg, M. Barnes, D.S. Cala, K. Guan, K.S. Hemes, et al. 2022. Informing Nature-Based Climate Solutions for the United States with the Best-Available Science. Global Change Biology 28:3778–3794.
Oregon Solar 1 Storage Industries Association. n.d. “Oregon Community Solar Program.” https://www.orssia.org/communitysolar. Accessed February 21, 2023.
Orvis, R., and M. Mahajan. 2021. “A 1.5°C NDC for Climate Leadership by the United States.” Energy Innovation. https://energyinnovation.org/wp-content/uploads/2021/04/A-1.5-C-Pathway-to-Climate-Leadership-for-The-United-States_NDC-update-2.pdf.
Pacala, S., and R. Socolow. 2004. “Stabilization Wedges: Solving the Climate Problem for the Next 50 Years with Current Technologies.” https://www.science.org/doi/10.1126/science.1100103.
Powell, T.W.R., and T.M. Lenton. 2012. “Future Carbon Dioxide Removal via Biomass Energy Constrained by Agricultural Efficiency and Dietary Trends.” Energy and Environmental Science 5(8):8116. https://doi.org/10.1039/c2ee21592f.
Read, Q.D., S. Brown, A.D. Cuéllar, S.M. Finn, J.A. Gephart, L.T. Marston, E. Meyer, K.A. Weitz, and M.K. Muth. 2020. “Assessing the Environmental Impacts of Halving Food Loss and Waste Along the Food Supply Chain.” Science of the Total Environment 712:136255. https://doi.org/10.1016/j.scitotenv.2019.136255.
Rinke Dias De Souza, N., B.C. Klein, M.F. Chagas, O. Cavalett, and A. Bonomi. 2021. “Towards Comparable Carbon Credits: Harmonization of LCA Models of Cellulosic Biofuels.” Sustainability 13(18):10371. https://doi.org/10.3390/su131810371.
Ritchie, H. 2017. “How Much of the World’s Land Would We Need in Order to Feed the Global Population with the Average Diet of a Given Country?” Our World in Data. October 3, 2017. https://ourworldindata.org/agricultural-land-by-global-diets.
Robertson, G.P., S.K. Hamilton, K. Paustian, and P. Smith. 2022. “Land-Based Climate Solutions for the United States.” Global Change Biology 28(16):4912–4919. https://doi.org/10.1111/gcb.16267.
Rosegrant, M.W. 2008. Biofuels and Grain Prices: Impact and Policy Responses. Washington, DC: International Food Policy Research Institute.
Santo, R.E., B.F. Kim, S.E. Goldman, J. Dutkiewicz, E.M.B. Biehl, M.W. Bloem, R.A. Neff, and K.E. Nachman. 2020. “Considering Plant-Based Meat Substitutes and Cell Based Meats: A Public Health and Food Systems Perspective.” Frontiers. https://www.frontiersin.org/articles/10.3389/fsufs.2020.00134/full.
Saunders, P.J. 2020. “Land Use Requirements of Solar and Wind Power Generation: Understanding a Decade of Academic Research.” Energy Innovation Reform Project. https://www.innovationreform.org/2020/10/12/land-use-requirements-of-solar-and-wind-power-understanding-a-decade-of-academic-research.
Schmer, M.R., K.P. Vogel, R.B. Mitchel, R.K. Perrin. 2008. “Net Energy of Cellulosic Ethanol from Switchgrass.” Proceedings of the National Academy of Sciences 105(2)464. https://www.pnas.org/doi/10.1073/pnas.0704767105.
Scully, M.J, G.A. Norris, T.M. Alarcon Falconi, and D.L. MacIntosh. 2021. “Carbon Intensity of Corn Ethanol in the United States: State of the Science.” Environmental Research Letters 16(4):043001. https://doi.org/10.1088/1748-9326/abde08.
Seddon, N. 2022. “Harnessing the Potential of Nature-Based Solutions for Mitigating and Adapting to Climate Change.” Science 376(6600):1410–1416. https://doi.org/10.1126/science.abn9668.
Seddon, N., A. Chausson, P. Berry, C.A.J. Girardin, A. Smith, and B. Turner. 2020. “Understanding the Value and Limits of Nature-Based Solutions to Climate Change and Other Global Challenges.” Philosophical Transactions of the Royal Society B: Biological Sciences 375(1794):20190120. https://doi.org/10.1098/rstb.2019.0120.
Shigeta, R. 2020. “Lab-Grown Meat Is Scaling Like the Internet.” The Spoon, October 26. https://thespoon.tech/lab-grown-meat-is-scaling-like-the-internet.
Smith, L.J., and M.S. Torn. 2013. “Ecological Limits to Terrestrial Biological Carbon Dioxide Removal.” Climatic Change 118(1):89–103. https://doi.org/10.1007/s10584-012-0682-3.
Stubbs, M., K. Hoover, J.L. Ramseur. 2021. “Agriculture and Forestry Offsets in Carbon Markets: Background and Selected Issues.” R46956. Washington, DC: Congressional Research Service. https://crsreports.congress.gov/product/pdf/R/R46956.
Swartz, E., A. Ravi, A. Reeber, J. Levink, T. Huang, and B. Smith. 2023. Anticipated Growth Factor and Recombinant Protein Costs and Volumes Necessary for Cost-Competitive Cultivated Meat. Washington, DC: The Good Food Institute.
Troya, M., H. Kurawadwala, B. Byrne, R. Dowdy, and Z. Weston. 2022. “Plant-Based Meat: Anticipating 2030 Production Requirements.” Good Food Institute. https://gfi.org/resource/anticipating-plant-based-meat-production-requirements-2030.
UNEP (United Nations Environment Programme). 2017. “Emissions Gap Report 2017.” http://www.unep.org/resources/emissions-gap-report-2017.
USDA (U.S. Department of Agriculture). 2014. “U.S. Forest Resource Facts and Historical Trends.” FS-1035. https://mff.forest.mtu.edu/TreeBasics/USFSfacts&trends2014.pdf.
USDA. 2017. “Major Land Uses: Summary Table 1: Major Uses of Land, by Region, State, and United States, 2012.” https://www.ers.usda.gov/data-products/major-land-uses/major-land-uses/#Summary%20tables.
USDA. 2023. “U.S. Bioenergy Statistics.” Economic Research Service. https://www.ers.usda.gov/data-products/u-s-bioenergy-statistics.
USGCRP (U.S. Global Change Research Program). 2018. Second State of the Carbon Cycle Report (SOCCR2): A Sustained Assessment Report, N. Cavallaro, G. Shrestha, R. Birdsey, M.A. Mayes, R.G. Najjar, S.C. Reed, P. Romero-Lankao, and Z. Zhu, eds. Washington, DC. https://doi.org/10.7930/SOCCR2.2018.
Vergeer, R., P. Sinke, I. Odegard. 2021. “TEA of Cultivated Meat: Future Projections for Different Scenarios.” CE Delft.
Waite, R., T. Searchinger, J. Ranganathan, and J. Zionts. 2022. “6 Pressing Questions About Beef and Climate Change, Answered,” World Resources Institute. https://www.wri.org/insights/6-pressing-questions-about-beef-and-climate-change-answered.
White, A.E., D.A. Lutz, R.B. Howarth, and J.R. Soto. 2018. “Small-Scale Forestry and Carbon Offset Markets: An Empirical Study of Vermont Current Use Forest Landowner Willingness to Accept Carbon Credit Programs,” C.T. Bauch, ed. PLOS ONE 13(8):e0201967. https://doi.org/10.1371/journal.pone.0201967.
White House Council on Environmental Quality, White House Office of Science and Technology Policy, White House Domestic Climate Policy Office. 2022. Opportunities for Accelerating Nature-Based Solutions: A Roadmap for Climate Progress, Thriving Nature, Equity, and Prosperity. Report to the National Climate Task Force. Washington, DC. https://www.whitehouse.gov/wp-content/uploads/2022/11/Nature-Based-Solutions-Roadmap.pdf.
Willett, W., J. Rockström, B. Loken, M. Springmann, T. Lang, S. Vermeulen, T. Garnett, et al. 2019. “Food in the Anthropocene: The EAT–Lancet Commission on Healthy Diets from Sustainable Food Systems.” Lancet 393(10170):447–492.
Williams, J.H., R.A. Jones, B. Haley, G. Kwok, J. Hargreaves, J. Farbes, and M.S. Torn. 2021. “Carbon-Neutral Pathways for the United States.” AGU Advances 2(1):21. https://doi.org/10.1029/2020AV000284.
Winiwarter, W., L. Höglund-Isaksson, Z. Klimont, W. Schöpp, and M. Amann. 2018. “Technical Opportunities to Reduce Global Anthropogenic Emissions of Nitrous Oxide.” Environmental Research Letters 13(1):014011. https://doi.org/10.1088/1748-9326/aa9ec9.
Wu, C., S.R. Coffield, M.L. Goulden, J.T. Randerson, A.T. Trugman, and W.R.L. Anderegg. 2023. “Uncertainty in US Forest Carbon Storage Potential Due to Climate Risks.” Nature Geoscience 16:422–429.
Yin, J., D. Yang, X. Zhang, Y. Zhang, T. Cai, Y. Hao, S. Cui, and Y. Chen. 2020. “Diet Shift: Considering Environment, Health and Food Culture.” Science of the Total Environment 719:137484. https://doi.org/10.1016/j.scitotenv.2020.137484.
YSG Solar. 2022. “3 Largest Floating Solar Farms in the United States in 2022.” https://www.ysgsolar.com/blog/3-largest-floating-solar-farms-united-states-2022-ysg-solar.
Zhou, Q., K. Guan, S. Wang, C. Jiang, Y. Huang, B. Peng, Z. Chen, et al. 2022. “Recent Rapid Increase of Cover Crop Adoption Across the U.S. Midwest Detected by Fusing Multi-Source Satellite Data.” Geophysical Research Letters 49(22). https://doi.org/10.1029/2022GL100249.